참고문헌
[1] 이차원 물질의 밸리트로닉스 연구, 류혜진 (DOI: 10.3938/PhiT.29.022)
[2] Electrical tuning of valley magnetic moment through symmetry control in bilayer MoS2, Wu et al, (DOI: 10.1038/NPJYS2524)
[3] Valleytronics in 2D materials. Schaibley et al, (DOI: 10.1038/natrevmats.2016.55)
[4] Wikipedia, Valleytronics
[5] Valleytronics: Opportunities, Challenges, and Paths Forward (DOI: 10.1002/smll.201801483)
[6] Y., Gao, Y., Zhang, S. et al. Valleytronics in transition metal dichalcogenides materials. Nano Res. 12, 2695–2711 (2019). https://doi.org/10.1007/s12274-019-2497-2
[7] Valley-dependent spin polarization in bulk MoS2 with broken inversion symmetry
0. Intro
* 해당 글은 제 개인적인 이해를 통해 작성되었으므로 틀린부분을 포함할 수도 있습니다.
전자의 자유도에는 작게는 두가지부터 크게는 네가지가 있다고 알려져있다.
이를 나열하면 다음과 같다.
- Electric Charge
- Spin
- Valley
- Orbital
현재 산업군에서 쓰고있는 전자의 자유도는 위 4개 중에서 전하만을 사용하는 것이다. 전하 뿐만 아니라 spin이라는 degree of freedom을 사용하는 것이 스핀트로닉스(spintronics), 전하의 valley degree of freedom을 사용하는 것을 밸리트로닉스(valleytronics), 마지막으로 orbital degree of freedom을 사용하는 것이 오비트로닉스(orbitronics)이다. 우리가 이 글을 통해 알아볼 것은 밸리트로닉스이다. (+ 여기서 말하는 valley는 conduction band가 가지는 계곡(valley)모양을 본따 만들어졌다)
1. Valleytronics Basic
반도체공학을 배우면 모를 수가 없는 두 단어가 있다. 바로 가전자대(valence band)와 전도대(conduction band)이다.
Valence band에 속박되어있던 전자는 외부에서 에너지를 받아 band gap을 뛰어넘어 conduction band로 이동한다. 이때 실제 고체의 경우 전자가 이동하는 concduction band의 minima는 한 에너지에 대해 여러개의 밴드가 존재하는 energy-degenerated가 형성되어 있다. 이는 아래 그림을 보면 이해할 수 있다.
그림에도 설명되어 있듯이, 특정 물질군에서는 같은 에너지에 대해 두개 이상의 energy band가 존재할 수 있다. 즉, 에너지가 degenerated된 것이다. 하지만 이와 동시에 crystal axes는 서로 다른 값을 가지므로 momentum vector는 다를 것이다.
다시 말하면, 특정 전자가 conduction band로 이동했을 때, energy-degenerated된 conduction band minima에서 전자를 '에너지'적으로 구분하는 것은 불가능하다(당연히 에너지 값이 모두 같으니까!). 하지만 conduction band가 놓여있는 crystal axes의 방향에 따라 전자가 가지는 crystal momenta가 달라진다. 이 서로 다른 물리량(crystal momenta)을 이용해 degree of freedom을 정의하고 이용하는 것이 우리가 말하고 있는 밸리트로닉스이다.
MRAM의 뇌피셜: 그렇기에 valleytronics에서 valley degree of freedom은 꼭 2개로 한정할 필요는 없다. k-space속 crystal momenta는 spin처럼 up, down으로 나눠지는 이산적인 수가 아니라 연속적인 벡터이므로 이론적으로는 무한대의 valley degree of freedom도 가능할 것이다.
기본적으로 valley state는 외력과 강하게 커플링 되어있지 않다. 다시 말해 외부에 전기장이나 자기장을 가해도, valley degree of freedom을 manipluation시키거나 측정할 수 없다는 것이다. 그러므로, 대부분의 물질은 유용한 밸리트로닉스 소자에 부적합하다. 이는 스핀트로닉스와는 반대되는 것이다. 스핀트로닉스에서는 전자의 스핀이 spin magnetic moment를 가지기 떄문에 자기장에 의한 manipulation이 가능하고, 또한 SOC를 통해 전기장을 이용해서도 스핀의 manipulation이 가능하다.
몇몇 물질 중에서는 서로 다른 crystal axes 방향에서, 질량의 anisotropy가 발생하여 외부장이 가해졌을 때 valley polarization을 유발할 수도 있다. 이러한 현상은 극 저온의 다이아몬드, AlAs, Si, Bi 등에서 관측된다. 하지만 이 물질들 역시 valley index와 외부장 사이에 강력한 coupling이 부재하므로 밸리트로닉스 소자로서는 역시 부적합하다.
하지만 특정 물질들에서는 valley degree of freedom이 잘 address 된다. 바로 그래핀, MoS2 monolayer 등의 물질이다.
이 물질들은 honeycomb lattice를 가지며, 이로 인해 K와 K'에서 energy degenerated 된 bandstructure를 가진다. 다시 말해 valley degree of freedom은 정의됨을 확인했다. 하지만 어떻게 address하는가? 이를 위해서는 서로 다른 states들을 구분할 실존하는 물리량이 필요하다. 이 물리량에는 Berry curvature와 orbital angular moment가 있다.
앞에서 언급한 MoS2, WSe2 등의 TMDS 물질은 monolayer, 또는 odd-number layer일때 TRS가 보존됨과 동시에 SIS가 깨져 Berry curvature가 발생한다. 이 Berry curvature는 인가되는 전기장과 crystal momenta를 coupling시켜 valley-selective interaction을 보인다.
(Berry curvature에 대한 자세한 내용은 베리 곡률이란 도대체 뭘까? 를 참고해주세요)
정리하면 다음과 같다.
Effects | Origin |
Defining valley defree of freedom | honeycomb lattic |
Manipulation of valley degree of freedom | Berry curvature (by inversion symmetry breaking) |
From valleytronics to spintronics | large SOC in the material |
2. Symmetry
바로 위에서 언급한 "TRS가 보존됨과 동시에 SIS가 깨져 Berry curvature가 발생한다."를 이해하기 위해서는 두가지 개념이 필요하다. 우선 첫번째로 Berry curvature가 pseudovector라는 것, 그리고 두번째로는 symmetry에 대한 개념이다.
2.1. Pseudovector
pseudovector는 두개의 서로 다른 vector가 cross product하여 만드는 vector이다. Symmetry에서 pseudovector가 중요해지는 이유는 아래 그림을 보면 쉽게 이해된다.
위 그림에서 3D 화살표는 모두 pseudovector이며, 그 화살표를 축으로 회전하는 원형고리는 실제 벡터들의 외적 방향이다. mirror plane transformation을 하게 되면 고리의 방향이 앞에 두개는 변하지만 맨 마지막은 변하지 않는다. 결과적으로 그림 속 앞 두개는 mirror plane transformation에 대해 대칭이 깨진 형태이며, 맨 뒤에있는 경우만 대칭이 성립한다고 할 수 있다. 즉, pseudovector를 대칭시킬 때는 vector 그 자체를 보는 것이 아니라, vector를 중심으로 그리는 원형 고리를 생각하면 된다.
xz 평면, 그리고 xy평면에 대한 mirror transformation은 x축으로 놓여있는 자화의 원형고리의 방향을 반대로 바꿀것이다. 결과적으로 transformation이후의 자화는 -x축을 바라볼 것이며 대칭은 깨진다.
2.2. TRS, SIS
TRS, SIS에 대해 물리량은 다음과 같이 작용한다.
When time-reversal symmetry holds,
When spatial inversion symmetry holds,
만약 system이 TRS와 SIS를 동시에 만족한다면, Berry curvature의 값은 0이 될 것이다. 즉, SIS가 깨져 system이 다음의 조건을 만족할 때 non-vanishing Berry curvature를 가지게 된다.
전기장 내에서 non-vanishing Berry curvature를 가지는 Bloch eletrons에 대한 semi-classical equation of motion은 다음과 같다.
이 식에서 보면 $\dot{\vec{k}}$은 전기장에 비례하기에 K와 K'에서 서로 다른 값을 갖지만,
여기서 Berry curvature $\vec{\Omega}$는 Bloch function을 이용해 정의할 수 있다.
여기서 $\vec{A}_{n}$은 Berry connection이고, $u_{n}$은 n번째 에너지 밴드에 있는 Bloch electron wavefunction의 periodic part이다. Berry curvature는 또한 아래와 같이 쓸 수 있다.
여기서 $E_{n}^{0}(\vec{k})$는 n번째 밴드의 energy dispersion이며, $\vec{P}_{n,i}(\vec{k})= \left< u_{n}|V|u_{i} \right> $는 velocity operator 행렬의 n행 i열 성분이다.
Equation of motion이 system의 symmetry에 대해 invariant하다는 것을 강제하면, TRS에서는 아래의 식이 성립할 것이고,
SIS에서는 아래의 식이 성립할 것이다. (Pseudo vector)
Equation of motion을 통해, 2D hexagonal crystal에 in-plane electric field가 작용할 경우 Berry curvature가 field에 수직한 방향으로 전자의 움직임을 만들어냄을 알 수 있다. 또한 이 전자의 움직임은 valley의 부호에 따라 서로 반대를 향할 것이다. 이를 valley Hall effect라 한다.
이때, 단층 TMDs의 경우 SIS가 깨져있다. (최근에는 h-BN기판과의 결합이나 수직 방향의 전기장 인가와 같이 ㅣㄴ위적으로 SIS를 깨드리는 실험적 방법들도 소개되고 있다). Broken inversion symmetry는 또한
구체적인 예시로서, 많은 2D TMDs의 2H phase는 inversion symmetry가 부재하고, 이에 따라 K와 K' valley에서 서로 반대되는 $\vec{\Omega}$, $\vec{m}$을 갖는다. K와 K' points 부근의 band edge에서 $\vec{k}\cdot \vec{p}$ Hamiltonian은 아래와 같이 주어진다.
여기서 a는 lattice spacing, t는 nearest-neighbor hopping integral, $\tau_{z}$는 valley index로 1, 또는 -1의 값을 가지는 수 이고 $\sigma$는 Pauli matrix element, 그리고 마지막으로 $\Delta$는 밴드갭이다.
이 경우, conduction band에서의 Berry curvature는 다음과 같이 주어진다.
두개의 서로 다른 valley에서 반대의 부호를 가지는 Berry curvature 때문에, in-plane 전기장은 캐리어에 VHE를 유발한다.
이때 valent band의 Berry curvature와 condcution band의 Berry curvature는 부호가 반대이지만 값은 같다는 점을 알아두자. Orbital magnetic moment는 valence band와 conduction band에서 아래와 같이 서로 같은 값을 갖는다.
Orbital magnetic moment가 0이 아닌 갑을 갖는다는 것은 valley가 상반되는 magnetic moments ($\tau_{z}= \pm 1 $)을 갖는다는 것이고, 그러므로 magnetic signature를 통해 valley polarization을 찾을 수 있다.
TRS가 유지됨에도 서로 상반되는 값을 가지기 위해서는 물질의 SIS 특성이 부재해야 한다.
A more accurate statement would be: "The Berry curvature can be thought of as analogous to a magnetic field in k-space, but it is not a magnetic field in the conventional sense."
3. 밸리 홀 효과 (Valley Hall effect)
단층 TMD 물질의 경우 서로 다른 밸리가 가지는 반대 부호의 베리 곡률에 의해 전류에 수직한 방향으로 K와 -K 밸리의 전자가 서로 반대 방향으로 transverse velocity를 얻는 것이다.
이러한 현상을 valley Hall effect라 하며, 외부 자기장 없이 순수하게 전기적으로 제어되는 밸리트로닉스를 가능하게 한다.
광학적 밸리 펌핑
단층 TMD는 벌집 격자(honey comb) 형태의 2차원 결정 구조를 가지는 물질로 밴드 구조를 살펴보면 운동량 공간(momentum space)의 서로 다른 두 지점에 에너지가 같은 K와 -K라는 두개의 밸리를 가진다.
에너지가 같은 이 K, -K 밸리의 전자를 각각 독립적으로 제어하기 위해서는 두 밸리를 구분지을 수 있는 물리량이 필요하며, 또한 이러한 물리량은 외부에서 측정 가능해야 한다.
이를 위해 K에 시간 역전 대칭을 취하면 -K가 된다는 점에 주목해보자.
이렇게 시간 역전을 취했을 때 크기는 동일하게 유지하되 부호만 바뀌는 물리량은, 시간 역전에 대해 홀수 패리티(odd parity)를 가졌다고 한다.
K와 -K 밸리에서 시간 역전 대칭에 대해 홀수 패리티를 가지면서 측정 가능한 물리량을 찾을 수 있다면 우리는 두 밸리를 서로 구분지어 제어하는 방법을 찾을 수 있을 것이다.
여기서 궤도 자기 모멘트(orbital magnetic moment, OMM)는 시간 역전에 대해 홀수 패리티를 가지는 물리량의 좋은 예이다.
다시 말해 K와 -K밸리의 전자는 서로 크기는 같지만 부호는 반대인 OMM을 가진다.
실험적으로 이 사실은 각각의 밸리의 전자를 특정 원평광을 가지는 빛을 사용해 선택적으로 여기시키는 광학적 밸리 펌핑이 가능함을 의미한다. 이로부터 TMD에서 빛을 사용한 다양한 방향의 밸리트로닉스 연구가 가능해졌다.
격자의 반전 대칭성
위에서 처럼 OMM과 베리 곡률을 활용하여 K와 -K 밸리의 전자를 각각 선택적으로 제어학 ㅣ위해서는 고려해야 할 점이 한가지 더 있다.
이는 물질의 반전 대칭 깨짐(inversion symmetry breaking)이다.
K와 -K는 공간 반전 대칭(spatial inversion symmetry)관계에 있는데, OMM과 베리 곡률은 공간 반전에 대해 크기와 부호가 바뀌지 않는 짝수 패리티(even parity)를 가진다.
→ 반전 대칭적인 물질에서는 궤도 자기 모멘트와 베리 곡률이 모두 0이 된다.
그러나, 단층 TMD와 같이 반전 대칭이 깨진 물질은 공간 반전 대칭에 의한 제약 조건이 없기 때문에 K와 -K 밸리에서 서로 반대 부호의 OMM와 베리 곡률을 가질 수 있다.
대조적인 예로, 그래핀이나 이중층 TMD의 경우에는 반전 대칭적인 격자 구조를 가지고 있어서 OMM과 베리 곡률이 0이고, 밸리에 의한 전자 제어가 원칙적으로 불가능하다.
그러나 최근에는 h-BN 기판과의 결합이나 수직 방향의 전기장 인가와 같은 방법으로 반전 대칭을 인위적으로 깨뜨리는 실험적 방법들이 소개되고 있어서 반전 대칭적인 물질을 활용한 밸리트로닉스도 많은 연구가 되고 있다.
연구 동향
2014년 = 빛
밸리 홀 효과에 대한 직접적인 관측은 optical Kerr rotation microscopy를 사용한 실험으로 이루어졌다.
이 실험에서는 선형 편광된 빛이 자성을 가지는 물질에서 반사될 때 선평광의 방향이 회전한다는 Kerr effect를 활용하여 K와 -K 밸리에 의해 물질에 생성된 밸리 편극(polarization)을 측정하였다.
특히 이중층 $MoS_{2}$트랜지스터의 경우 본래 반전 대칭을 가지고 있는 물질이지만 수직 방향의 게이트 전압을 사용하면 반전 대칭을 깨뜨림과 동시에 밸리 홀 효과의 크기와 부호를 스위칭 하는 것이 가능하다.
전기적으로 밸리를 제어하는 또 다른 방법은 밸리 자기전기성(magnetoelectricity)을 활용하는 것이다.
자기전기성은 전기적으로 물질의 자화(magnetization)를 유도하거나 반대로 자기장으로 물질에 전기적 펴극을 유도하는 현상을 말한다.
그러나 단층 TMD의 경우 3중 회전 대칭성으로 인해 자기전기 현상이 일어나지 않는데, 결정을 한 방향으로 늘어뜨리는 변형(strain)을 가하면 이 회전 대칭성을 깨뜨릴 수 있다. 이때 물질에 듀호된느 밸리 자화는 단층 면에 가하는 전기장의 방향과 크기를 통해 제어된다.
그림 3은 방사형으로 전류가 흐르는 원형 전극을 사용해서 물질의 밸리 자화가 전기장의 방향에 따라 변화하는 모습을 보여준다. 이러한 밸리 자기전기 현상은 비휘발성 메모리 소자에 활용될 수 있기 때문에 벨리트로닉스 응용에 있어서 높은 기술적 가치를 가진다.
최근에는 단층 TMD 물질에 변형을 가하면 베리 곡률의 비대칭적인 분포에 의해 베리 곡률 쌍극자 (Berry curvature dipole)를 유도할 수 있다는 사실이 밝혀지면서 물질의 변형이 밸리트로닉스 구현의 한가지 가능성으로 제시되었다.
베리 곡률 쌍극자는 양자 비선형 홀 효과(quantum nonlinear Hall effect)를 일으키는 등 고체 물질에 다양한 양자 기하학적 현상을 발생시킬 것으로 기대되는 물리량이다.
2019년에는 $MoS_{2}$에 변형을 자유롭게 가할 수 있는 유연 소자로부터 베리 곡률 쌍극자를 직접 측정함으로써 밸리 자기전기 현상의 미시적 메커니즘을 베리 곡률 쌍극자로 이해할 수 있게 되었다.
ㅡㅡ
Abstract
블로흐 전자가 첫번째 브릴루앙 존에서 가지는 valely degree of freedom은 양자 컴퓨팅 및 정보 저장 분야에 혁신적인 접근법을 제공한다. Time-reversal symmetry(TRS)의 존재와 더불어 spatial inversion symmetry(SIS)의 부재는 블로흐 전자가 0이 아닌 베리 곡률과 궤도 자기 모멘트를 갖게끔 하며, 이 두 물리량을 통해 valley Hall effect와 밸리트로닉스에서의 optical selection rules을 구현할 수 있다. 더욱이, 최근 뜨고있는 transition metal dichalcogenides (TMDs) 물질들은 그들이 내재하고 있는 구조적, 광학적, 그리고 전기적 특성 덕분에 밸리트로닉스 연구에 있어서 이상적인 후보군으로 점처지고 있다. 특히 TMDs monolayer에서의 direct bandgap과 SIS의 부재가 그중에서도 그러하다. 하지만 inter-valley relaxation에 대한 메커니즘은 아직 완벽히 해결되지 않았으며, valley manipulation에 대한 복잡성은 밸리트로닉스 소자의 실현을 가로막고 있다. 해당 리뷰에서, 저자는 체계적으로 valley degree of freedom의 근본적인 특성과 tuning 전략 (광학적, 전기적, 자성적, 그리고 메카니컬한 튜닝 등)을 다룰 것이며, 최근의 TMDs 기반 밸리트로닉스 소자 진행 상황을 요약할 것이다. 저자는 또한 현재의 문제점과 가능성에 대해 다루며 결론을 내릴 것이다.
1. Introduction
New degrees of freedom possess the immense potential and attract huge attentions as the imminent end of “Moore's Law”. 전통적인 charge degree of freedom과 비교하여, 스핀은 응집 물질 전기학에서 발현되는 추가적인 internal degree of freedom이며 높은 집적도, 빠른 처리속도, 낮은 파워소모, 그리고 비휘발성등을 가지는 스핀트로닉스 소자를 가능케 한다. 더욱이 최근에, 여러 crystal들(Si, Ge, Bi, diamond, GaAs, graphene, silylene, 그리고 TMDs)에서 전자가 추가적인 internal degree of freedom, 다시 말해 valley, 을 가질 것이라는 것이 예측됐다. Valley는 crystal의 momentum space에서의 극값을 뜻하며, conduction band의 극소값과 valence band의 극대값의 국소적 영역이라 할 수 있다. 밸리트로닉스 응용을 실현시키기 위한 기본적이자 주요한 principle은 서로 다른 valley에서 carrier가 다른 양을 가지며 비등가적으로 분포하는 것(valley polarization)이다. Valley polarization은 SIS가 부재한 시스템에서 컨트롤이 가능하다. TMDs는 monolayer일때 SIS가 부재한 물질로 밸리트로닉스에 적절한 물질이라 할 수 있다. Valley degree of freedom을 tuning하고 detecting하는 거슨 마이크로-나노 레벨에서의 정보 manipulation과 encoding에서 매우 훌륭한 potential을 제공할 것이다. 밸리트로닉스를 기반으로 하여, 다양한 기능의 양자 소자와 양자 컴퓨팅을 실현시킬 것으로 기대된다. 하지만, 밸리트로닉스 활용에는 수많은 도전과제들이 놓여있다. Valley depolarization의 메커니즘은 아직 해결하지 못한 숙제이며, 밸리트로닉스 소자의 응용응 가로막는 중심 포인트는 바로 TMDs 속 degree of valley polarization이 상온에서 매우 급격하게 감소하기 때문이다. 이는 phonon-determinating inver-valley scattering 때문이다. 게다가 간단하면서도 편리한 방법 (이를테면 광학적, 전기적, 자성적, 그리고 메카니컬한 튜닝)으로 외부에서 valley polarization을 규제하는 것 역시 중요하다. 다른 한편으로, valley exciton의 상대적으로 짧은 수명도 실용적인 밸리트로닉스 활용과 효율적인 valley의 manipulation을 어렵게 한다. 최근에, 2D heterostructure를 개발하여 valleytronics에 이용한 연구진도 이었다. By assembling different 2D materials, the lifetime of valley excitons is expended to μs order of magnitude. And with the help of magnetic materials, valley polarization is easier to be realized [32]. Additionally, in the heterostructure device encapsulated by hexagonal boron nitride (h-BN), degree of valley polarization is relatively high [33]. Therefore, TMDs materials and the relative heterostructures provide a platform for the research and application of valleytronics. In this review, we focus on the properties of the valley, tuning methods and valleytronics devices in 2D materials and provide our conclusion of present challenges as well as the outlook on further research.
2. The valley properties
2.1 Valley degree of freedom of electrons
Although the current research in valleytronics is mainly based on TMDs, this concept is originally derived from the Dirac fermions in graphene. Graphene has a hexagonal honeycomb structure containing two non-equivalent carbon atoms [34, 35], which are conventionally referred to as A sub-lattice and B sub-lattice (Fig. 1(a)). The nearest neighbors to the atoms on the A site are three atoms on the B site. The vector is d1,2 = (± 3 x + y)d/2 and d3 = −dy, where d is the nearest neighbor distance. By inverting the three vectors dj (j = 1, 2, 3), we get three vectors from the B site to the nearest neighbor atom on the A site. The lattice vector between the nearest neighbor atoms connecting the same set of lattice points is a1,2 = (± 3 x + 3y)d/2. The corresponding inverted vector is b1.2 = (± 3 x + 3y)/3d, so the first Brillouin zone is hexagonal. In the hexagonal Brillouin zone, there are two different points that are linked by time-reversal symmetry, ±K = (±4π/3a)x (Dirac point). The other corners of the Brillouin area are connected to ±K by inverted lattice shift. By calculating the quasi-particle dispersion relation in graphene, the dispersion relation spectrum is obtained (Fig. 1(b)). At the six Dirac points (+K and −K), the valence band and the conduction band are in linear contact, which means zero band gap in graphene (Fig. 1(c)). +K and −K valleys are inequivalent but degenerate in energy. The two quantities, Berry curvature ( ( )) Ω k n and orbital magnetic moment ( ( )) m k , are fundamental of the valleytronics. One of the important manifestations of Bloch’s electron related to valley properties is the Berry curvature, which is equivalent to an effective magnetic field in the momentum space [38]. When an in-plane uniform electric field E is applied in the system without inversion symmetry, the velocity of electrons is expressed as
here, ( ) v k n is the speed of electron. ( ) nε k is the energy in the momentum space. e is the elementary charge. E is the external electric field. ( ) Ωk n is the Berry curvature of Bloch electron. Obviously, in addition to the normal group velocity, the electrons also have an anomalous velocity associated with the Berry curvature, as shown in Eq. (1). Therefore, the Berry curvature can be regarded as an effective magnetic field, causing the electrons to deflect and resulting in transverse current and Hall conductance [39]. However, in a system with spatial inversion symmetry, considering the inversion symmetry and the time-reversal symmetry simultaneously, the Berry curvature can only be zero. The electrons will not have an anomalous velocity, so the broken inversion symmetry is necessary for valleytronics. For a system with broken inversion symmetry, the Berry curvature of the conduction band is shown in Fig. 1(d) (the Berry curvature of valence band has opposite signs, which is not shown). The Berry curvature at +K and −K valleys has opposite signs due to time-reversal symmetry [37]. Therefore, the electrons at +K and −K valleys will deflect in the opposite direction under the action of the in-plane electric field (Fig. 1(f)), which is the so-called valley Hall effect. Besides the spin magnetic moment, Bloch electrons carry an orbital magnetic moment [36], which exists accompanying the non-zero Berry curvature. It originates from the self-rotation of the wave packet. In the case of inversion symmetry breaking, the orbital magnetic moment of +K and −K valleys is equal in magnitude and opposite in sign (Fig. 1(e)), similar to Berry curvature. It is closely related to the optical selection rule in valleytronics. Since one of the applications of valleytronics is the field in information storage, realizing valley polarization is prerequisite. Since these energy valleys are separated largely in the momentum space, the transition of electrons between these discrete valleys requires the participation of phonons or the assistance of impurities to satisfy the conservation of momentum, which makes the transition very difficult. Therefore, the valley can be regarded as the inherent discrete degree of freedom of electrons or holes. The valley degree of freedom has a huge potential in future electronic devices because it provides a new way of encoding information. It is a promising approach to expand the data capacity to use all three intrinsic degrees of freedom to store information (charge, spin, and valley as shown in Fig. 1(g)), which will cause the performance of the next generation of electronic products is staggering.
2.2 Valleytronics in TMDs
The key to realizing the application of the valleytronic material is the generation of broken inversion symmetry on the honeycomb lattice. However, in graphene, the controllable staggered lattice potential is experimentally difficult to achieve. Recently, TMDs such as MoS2 have attracted much attention [29, 40–49]. The structure of the TMDs is similar to that of the honeycomb lattice, and its monolayer has natural broken inversion symmetry and direct band gap, which is proved to be an ideal valleytronic material. Taking MoS2 as an example, monolayer MoS2 consists of a monolayer of molybdenum atoms sandwiched between two layers of sulphur atoms in a trigonal prismatic structure (Fig. 2(a)). Different layers of the bulk MoS2 are bonded by van der Waals force. Interestingly, the bulk MoS2 has an indirect band gap and the band gap is relatively narrow. But as the number of MoS2 layers decreases, the band gap exponentially increases and reaches the limit of 1.8 eV in monolayer [50]. And the indirect bandgap changes to direct bandgap (Fig. 2(b)). According to the normalized photoluminescence (PL) spectra of MoS2 in different layers measured by Andrea Splendiani et al., the luminescence intensity in monolayer MoS2 is obviously larger than the few-layer and bulk MoS2 (Fig. 2(c)). That indicates that the luminescence quantum efficiency reaches the highest in monolayer MoS2 due to the direct band gap nature. Originating from its inherently broken inversion symmetry, its optical transition rule is expected to present a circularly polarized light selectivity. According to the calculation of the monolayer MoS2 conducted by Ting Cao et al. based on the density functional perturbation theory, when the spin-orbit coupling effect is not considered, the valley polarization of the direct transition between the top of the valence band and the bottom of the conduction band is uniformly distributed around the +K and −K valleys [51]. While at the boundary of the two valleys, the sign of valley polarization reverses (Fig. 2(d)), which means the transition of the electronic states in +K and −K valleys always couples with specific circularly polarized light (optical selection rules in valleytronics, illustrated in Fig. 2(e)). The valley polarization is specified by the following formula.
here, cv P k ( ) is the transition matrix element of circular polarization between conduction band and valence band. This provides a theoretical basis for the generation of valley polarization, which is a prerequisite for the application of valleytronics devices.
2.4 Hall effect in valleytronics
Hall effect devices play a significant role in sensors and switching devices. Quantum Hall effect can be used to control the information flow, such as spin and valley. Interestingly, carriers in ±K valleys deflect due to non-zero Berry curvature in the presence of longitude electric field which is so-called valley Hall effect. More importantly, the external magnetic field is not necessary for valley Hall effect devices. Therefore, it has a broad application prospect. Although it is a long time since valley Hall effect was proposed, distinct valley Hall voltage is measured experimentally in recent years [62–64]. Generally, the carrier density of +K and −K valley is equal. Valley Hall voltage is difficult to be detected because transverse charges cancel each other out. So, valley Hall voltage is unable to be detected. Therefore, to realize valley polarization by certain means such as optical injection is necessary. K. F. Mak et al. measured valley Hall voltage in monolayer MoS2 device under optical excitation [65]. As illustrated in Fig. 4(a), under optical circularly polarized excitation at 1.9 eV, a longitude electric field is applied. Electrons and holes in +K (or −K) valley increase and deflect to both sides of the device. Then distinct valley Hall voltage can be detected. And the Hall signal intensity increase as the longitude electric field increase (Fig. 4(b)). On the contrast, under linearly polarized excitation, valley Hall voltage is unable to be detected. The reason is that linearly polarized excitation leads to a simultaneous increase of carrier density of +K and −K valley. Valley polarization is not realized. After that, an identical experiment was conducted in bilayer MoS2 under circularly polarized excitation at 1.9 eV. As expected, there was no valley Hall voltage to be detected. The reason is that the inversion symmetry is recovered in bilayer MoS2. In consideration of spatial inversion symmetry and time-reversal symmetry, the Berry curvature is zero and valley Hall effect disappears. Generally, a longitude voltage is required as a driving force for carriers in Hall effect. However, Masaru Onga et al. conducted a Hall effect experiment of excitons (exciton Hall effect) in which the longitude voltage is not required [66]. In the exciton Hall effect, a circularly polarized light is applied on one end of the long and narrow device (Fig. 4(c)). The density of exciton in this endpoint increases rapidly and excitons flow to the other endpoint because of the gradients of the temperature and/or exciton density. Meanwhile, excitons in +K and −K valley deflect to both sides of the device due to the opposite Berry curvature but without breaking the bound states. Then excitons in both sides of the device recombine and emit different circularly polarized light. As a matter of fact, separated light emitting is difficult to be detected because the lifetime of excitons is short. As illustrated in Fig. 4(d), a linear polarized light at 1.96 eV is applied to generate excitons in +K and −K valley in a long and narrow monolayer MoS2 at 30 K. As the diffusion distance increases, the luminescence gets weaker. Simultaneously, the luminescence peak splites into two peaks on a spatial scale of 2 μm and the circular polarization is obvious (Fig. 4(e)). Subsequently, circularly polarized light is applied to generate valley-polarized excitons and selective spatial transport of valley-polarized excitons is realized (Fig. 4(f)).
3 Valley tunability
Since valleytronics is expected to be applied in information storage devices, it is of great importance to find specific experimental methods for tuning the valley degree of freedom. Electrical and magnetic methods are widely used in electronic devices. And the valley degree of freedom is also optically controllable based on optical selection rules in valleytronics. All these tuning methods are possible to achieve valley polarization.
3.2 Electrical tuning.
The electrical tuning of monolayer MoS2 has been explored by K. F Mak et al., the bias-dependent valley Hall voltage and gate-dependent Hall conductivity have been observed [65]. On the other hand, more abundant examples of effective electric modulation are realized in bilayer samples or pn junction devices (see section 5). Especially in bilayer TMDs materials, the selective optical absorption of circularly polarized light is forbidden in but allowed in monolayer, because the monolayer TMDs system has inversion asymmetry but bilayer TMDs system has inversion symmetry. Therefore, breaking inversion symmetry is the key to reach valley polarization in bilayer TMDs. The progress in electrical control of electronic properties in TMDs laid the foundation for electrical tuning of the valley [70–75]. Sanfeng Wu et al. developed an electrical method to continuously tune the inversion symmetry in bilayer TMDs [76]. As an electric field in the direction out of the plane was applied on bilayer MoS2, the potential difference between the upper and lower layers emerged gradually. Therefore, the inversion symmetry was broken (Fig. 6(a)). Under the left, circularly polarized excitation at 30 K with the 0 V gate voltage, the circular polarization of PL spectrum of bilayer MoS2 was about 0.1–0.3. It indicates that bilayer MoS2 prepared in the laboratory is not an ideal inversion symmetry system. The inversion symmetry is somewhat broken, which is consistent with the previous reports. When the gate voltage is applied to the material (as a vertical electric field), the degree of polarization of PL as a function of wavelength and gate voltage is shown in Figs. 6(c) and 6(d). Under the gate voltage of −60 V, the PL polarization is almost zero. It indicates that the sample is initially electron-doped. When the gate voltage was −60 V, it was intrinsic and the system reached inversion symmetry. As the gate voltage increased, the inversion symmetry was broken gradually. The PL polarization increased, and it tended to be saturated when the gate voltage was up to −30 V. For monolayer MoS2, the degree of PL polarization remained around 0.6 under different gate voltages (Fig. 6(e)). The reason is that the system has inversion asymmetry in monolayer MoS2. It shows the selective optical absorption of circularly polarized light by different valleys. According to their density functional theory (DFT) calculation, the reason for electrical tuning valley polarization is that the orbital magnetic moment is adjusted by the external electric field (Fig. 6(f)). On the other hand, a more intuitive measurement of valley magnetic moments was carried out through Kerr rotation measurement under certain gate voltages [77]. The gate voltage applies a perpendicular electric field and breaks the inversion symmetry present in bilayer MoS2. Because of the absence of inversion symmetry, electrons at the +K and −K valleys of Brillouin zone possess finite but opposite Berry curvatures. Therefore, electrons at the +K and −K valleys flow into the opposite edges of the device driven by the longitude electric field. The accumulation of electrons at the +K and −K valleys causes magnetic moments at both edges of the device which can be measured with Kerr rotation microscopy (Fig. 6(g)). As shown in Figs. 6(h)–6(j), when the gate voltage is 20 V (providing the vertical electric field), the inversion symmetry is broken. The valley electrons are deflected due to the non-zero Berry curvature and then accumulate at both edges of the device. It is apparent that a clear Kerr angle signal can be detected at both edges of the device, which indicates the accumulation of valley magnetic moment at both edges of the device. Similarly, when the gate voltage is −5 V, the Kerr angle signal at both edges of the device is obvious, but the sign of the Kerr angle is the opposite. However, when the gate voltage is 4 V, the Kerr angle signal is almost zero, which indicates no accumulation of valley magnetic moment. This confirms that 4 V is required to bring a bilayer MoS2 device to inversion symmetry because of the spontaneous doping of the bilayer MoS2 from the Si substrate. This phenomenon has been widely recognized for atomically thin samples on Si substrates.
6 Conclusion and outlook
In summary, we provide an overview of the properties of valley degree of freedom, development of tuning methods and quantum devices in valleytronics. Both theoretical and experimental advances have been made in valleytronics in recent years, especially the development of optical, electrical, magnetical and mechanical tuning methods of valley information. Nevertheless, there are still limitations impeding the scalable application of valleytronics. Firstly, as the performance of valleytronic devices strongly relies on the quality of samples, the controllable preparation of high-quality TMDs in large-scale is necessary for application. The one is the lack of practical tuning systems. So far, the optical and magnetic tuning is costly and sometimes needs a rigorous environment such as low temperature and vacuum. And mechanical tuning is unviable up to now due to the complex strain setup. While the electrical appliance is mature and well developed in micro-nano level. Therefore, all-electrical methods to initialize and read out valley information are vital for the application of valleytronics. Another key problem is the short valley lifetime. Short valley lifetime limits the length of valley transport and obstructs the progress in the valleytronic quantum device. Therefore, to prolong the valley lifetime is necessary for the application of valleytronics. Typically, assembling van der Waals heterostructure with different TMDs material layers or encapsulating with h-BN is regarded as an effective way to reduce the consumption of carriers and prolong the valley lifetime. In addition, the presence of spontaneous valley polarization has been expected in the ferrovalley materials such as VSe2 (in analogy to ferroelectric materials with spontaneous charge polarization and ferromagnetic materials with spontaneous spin polarization) [110]. With ferrovalley materials, it is possible to realize the simple tuning of valley polarization and the extension of valley lifetime. Hence, construction of 2D heterostructure and exploring new materials are necessary for further research of valleytronics. In summary, many novel physical mechanisms of valley degree of freedom deserve in-depth investigation and devices based on valleytronics are the candidates for the next generation information devices. The future of TMDs valleytronics is full of massive promising possibilities.
ㅡㅡㅡㅡ
전자의 valley 자유도는 다양한 물질 (그래핀, 다이아몬드, TMDs 등)에서 정보의 수송자로서 이목이 집중되고 있다. 이 중 monlayer TMDs 는 반도체적 특성을 가지며 상대론적인 SOC에서 유래하는 spin과 valley 사이의 coupling 때문에 독특한 특성을 가진다. 이 논문을 통해, 저자는 대표적인 TMDs인 MoS2에서 spin-ARPES, 그리고 ARPES를 통해 valley-dependent out-of-plane spin polarization을 direct observation한다. 이 결과는 first-principles 계산을 통한 이론적 예측과 일치한다. 이는 3R polytype crystal을 선택함으로써 가능해졌는데, 해당 물질은 non-centrosymmetric structure를 가진다. 이는 기존의 centrosymmetric 한 2H form과는 다른 것이다. 저자는 또한 3R form에서 circularly polarized photoluminescene spectrocopy를 통해 robust valley polarization도 확인했다. Non-centrosymmetric TMDs 는 spin/valley 자유도에 대한 자성적, 전기적 manipulation의 발전에 단단한 기반이 될 수 있을 것이다.
TMDs 기반의 원자 결정들은 다양한 물리적 특성(훌륭한 FET성능 with large on-off ratio and an ambipolar mode, electric field-induced superconductivity, Zeeman splitting, strong photoluminescene)을 가지는 떠오르는 물질이다. 특히 circular dichoic photoluminsecene은 'Valleytronics'라 불리는 optoelectronic functionality에 새로운 기회를 제공하는데, 이는 valley degree of freedom을 정보 수송자로서 사용하여 manipulation한다.
이 valleytronic의 후보 물질로는 실리콘, 다이아몬드, AlAs, Bi, 그래핀, 그리고 TMDs 등이 있다. Valley polarization의 control과 detection은 monolayer MoS2의 optical pumping과 다른 TMDs
Valley polarization의 control과 detection은 monolayer의 MoS2에서 optical pumping을 통해, 그리고 다른 TMDs 들에서는 photoluminescence circular dichroism에 의해 시연되었다. 이는 해당 물질의 유한한 bandgap을 이용한 것으로, 그래핀에서는 부재한 것이다. 이 특성은 monolayer MoS2의 non-centrosymmetric한 성질에서 비롯한 것으로 유한한 Berry curvature를 야기한다.
Fig 1a에 나와 있듯이, monolayer는 S-Mo-S가 준2차원적인 망을 만들며 공유결합을 통해 형성된다. 이를 통해 inversion symmetry 없이도 trigonal structure를 가지게 된다. 벌크에서는 이러한 2차원 monolayer는 약한 van der Waals 힘에 둘러 쌓인다.
MoS2의 여러 phase 중 bulk 에서 가장 대중적인 phase는 2H polytype으로, bilayer with space group P63/mmc로 구성된 유닛셀을 가지며, hexagonal 모양의 Briullouin zone을 가진다. 그리고 bulk crystal에 inversion symmetry를 가지고 있다.
TMDs를 unique하게 만들어주는 요소는, 중금속의 d orbital 때문에 발생하는 강력한 spin-orbit interaction (SOI) 때문인데, 이를 통해 spin physics와 valley physics를 이어준다. 이론적인 예측으로는 2차원 hexagonal Brillouine zone의 코너(K and K' points)에 위치한 가장 바깥 valence band가 undergo a valleycontrasting spin splitting with polarization perpendicular to the two-dimensional plane. Inversion symmetry의 부재 때문에, monolayer MoS2와 다른 TMDs는 spin/valley physics, 그리고 이를 넘어서는 전자, 혹은 광자소자에 가장 적합하다. 따라서, monlayer 샘플을 만드는 것이 키 포인트 이며 monlolayer 물질, 또는 소자의 특성과 제작에 엄청난 노력이 따르고 있다. monlayer 물질을 만드는 주된 방법으로 물리적 single crystal exfoliaion, CVD등이 사용되고 있으며 lateral 사이즈로 최대 30um의 crystalline monolayer를 보통 얻을 수 있다. 이 정도의 사이즈라면 기본적인 마이크로 사이즈의 소자를 통한 전기적 수송과 광학적 실험을 하는데 충분히 큰 사이즈이지만, spin/valley physics와 더 다양한 특성들에는 충분치 않을 수도 있다. 예를 들어, 이론적으로 예측되는 spin polarization은 아직 발견되지 않았다. 하지만, noncentrosymmetric structure는 꼭 monolayer에 한정될 필요는 없다.
최근에, bilayer 뿐만 아니라 심지어 bulk crystal에서의 symmetry control이 FET configuations을 통해 제안되고 있다. 전기장을 bilayer, 또는 multilayer에 수직으로 인가하는 것은 layer를 inequivalent하게 만들며; 결과적으로 in-plane inversion asymmetry가 다시금 생겨난다. 이러한 외부장을 이용한 crystal symmetry의 manipulation은 screening length 내에서의 surface state의 electronic Bloch state를 조절하는데 매우 필수적이며, monolayer state를 흉내내는데 새로운 방법을 제시한다.
이 논문에서 Here, we report a totally different way of controlling the symmetry by taking advantage of the rich polytypism in layered TMDC materials systems. In MoS2, as well as the most stable 2H phase, the so-called 3R phase (with the stacking pattern and first Brillouin zone shown in Fig. 1b,c, respectively) is known to exist as another stable phase28,29,34. The space group of the 3R structure is R3m, and its unit cell is composed of a trilayer stacked in such a way that the inversion symmetry is kept broken in the bulk form. Using single crystals of the 3R phase we successfully observed out-of-plane spin polarization in the valence band at the K and K′ points, in fair agreement with the first-principles band calculations, taking the SOI into account. This is proof of the valley-dependent spin polarization expected in monolayer MoS2, using the multilayer crystal with a non-centrosymmetric stacking pattern of layers.
We also demonstrate that information in the valley polarization in the 3R stacking is effectively preserved, by means of the layer number dependence of photoluminescence circular dichroism.
ㅡㅡㅡㅡ
Abstract
Inversion symmetry의 부재와 더불어 time-reversal symmetry의 존재는 2D TMDs에게 momentum space의 첫번째 브릴루앙 존 속 K, K' 포인트에 개별적으로 addressable한 valley를 부여한다. 이 valley addressability는 electron, hole, exciton의 momentum state를 이용하여 정보 처리에 새로운 패러다임을 열 수 있다는 가능성을 제시한다. 현대의 (혹은 양자의) information processing applicaion을 위한 valley degree of freedom의 manipulation과 관련된 기회들과 도전들은 2017년 Valleytronic Materials, Architectures, and Devices라는 워크숍에서 이미 제시된 바 있다. 이 리뷰 논문은 해당 워크숍에서 발표된 주요 내용들을 주제로 한다.
1. Background
The Valleytronics Materials, Architectures, and Devices Workshop, sponsored by the MIT Lincoln Laboratory Technology Office and co-sponsored by NSF, was held in Cambridge, MA, USA on August 22–23, 2017.
Valleytronics는 최근 이목을 끄는 분야로, 입자의 momentum index를 이용하여 (스핀, 전하와 함께) 정보 처리에 있어 획기적인 발전 가능성을 제시한다. 그래핀, TMDs와 같은 2D 물질을 isolation하는 것은 실험을 가능케 했고, valley physics에 대한 우리의 이론들을 확인시켜줬다.
하지만, 밸리트로닉 컴퓨팅이나 다른 기술들을 위한 유용안 디바이스의 발전은 물질의 퀄리티, 소자 디자인, 그리고 회로 아키텍쳐들의 혁신 없이는 요원해 보인다. 해당 워크숍에는 밸리트로닉스 분야에 내로라하는 연구자들이 모여 그들의 최신 연구 성과를 발표하였고, 밸리트로니스의 발전에 대한 기회와 문제점을 오픈 마인드로 논의 하였다. 세개의 상호적인 working session이 열렸으며, 정보 처리와 광공학 소자에 있어서의 잠재성 있는 응용부터 주요 미해결 물리학 문제 해결까지 다양하면서도 어려운 주제들에 대해 씨름했다.
이 article은 밸리트로닉스 소자의 잠재적 이점, 최신 밸리트로닉스 연구 동향, 그리고 이를 위해 뛰어넘아야 할 어려움들에 대해 독자들에게 알리기 위한 목적으로 작성됐다. 저자는 이 문서가 또한 미래의 정부 지원 연구 사업에 있어 올바른 방향으로 나아가는데 일조가 되길 바란다. 비록 해당 article에서 valley physics에 대한 소개와 현 지식의 상황을 제공하기는 하지만, 해당 문헌들의 포괄적인 리뷰를 위해 작성되지는 않았다. For that the reader is referred to several excellent review articles related to 2D materials and valleytronics
2. Introduction
원자들이 모여 결정을 형성할 때, 원자 속 전자들은 다른 전자들, 그리고 원자들과 상호작용을 하며 crystalline material의 전기적 특성을 결정하는 distinct bands를 만들어낸다.
반도체 crystal의 경우, the bonding electrons populate a filled band of allowed states known as the valence band, and are separated from an unfilled band of higher energy known as the conduction band by an energy gap that contains no allowed states (the band gap).
몇몇 반도체에서는 최소에너지의 영역이 conduction band에 생길 수도 있는데, that are indistinguishable from one another except for the direction of the crystal axes along which the energy band is oriented.
그러므로, 캐리어들이 valence band로 부터 band gap을 뛰어넘어 conduction band의 minima로 여기됐을 때, 서로 같은 에너지를 갖게(이를 energy-degenerate라 한다) 되지만, crystal momenta는 axes의 방향에 따라 다른 값을 가지게 될 것이다. 이러한 minima를 valley라 하며, 입자(electron, hole, exciton, etc..)들이 한개의 valley, 또는 서로 다른 valley에 있다는 점을 이용하는 소자들을 밸리트로닉스 소자라고 부른다. 특정한 momentum-distinguishable valley를 이 반대의 것에 대비하여 선택적으로 형성(populate)시키는 것—creating a valley polarization—이 밸리트로닉스를 가능케하는 열쇠이다.
입자들을 momentum space의 영역으로 localization하는 것은 valley pseudospin이라는 새로운 인덱스를 얻게끔 한다. 이는 일반적으로 입자와 연관된 양자화 스핀 인덱스처럼, 새로이 추가 되어지는 물리량이다. 비록 수많은 periodic 고체에서 에너지가 축퇴된 valley가 존재하지만, 일반적으로 특정 한 valley속 전자를 다른 valley에 속해있는 전자와 구별지어 따로 manipulate 하는 것은 불가능하다.이는 입자의 valley state가 외력과 강하게 커플링 되어있지 않기 때문이다. 그러므로, 대부분의 물질은 유용한 밸리트로닉스 소자에 부적합하다. 이는 스핀트로닉스와 반대되는 것인데, 스핀트로닉스에서는 전자의 스핀이 spin magnetic moment를 때문에 자기장에 의한 manipulation이 가능하고, 전기장 또한 SOC를 통해 스핀의 manipulation이 가능하기 때문이다. 또한 valleytronics가 유용해지려면 valley를 populating 하는 입자들이 관측이나 다른 원하는 행동을 할 때까지 머무르는 것 역시 매우 중요하다.
몇몇 물질에서는, 서로 다른 crystal 방향에서의 입자의 질량에 대한 anisotropy가, 외부장이 가해 졌을 때 valley polarization을 유발할 수도 있다; preferential scattering occurs from one valley to another. 이러한 현상은 극 저온의 다이아몬드, AlAs, Si, Bi 등에서 관측된다. 하지만, 이 물질들은 valley index와 외부장 사이에 강력한 coupling이 존재하지 않는다. 그러므로 특정 valley에 있는 particle에 대한 선택적 initialization, manipulation, 그리고 readout이 모두 불가능하다. 그렇기에 위 물질들은 valleytronics에 대한 논의에서 배제된다.
운 좋게도, valley pseudospin이 잘 address 되는 여러 물질들이 여럿 존재한다. 다른 물질들과는 (극명하게) 반대로, 그래핀, ���2 monolayer 같은 2D 물질은 Brilloun zone의 서로 다른 K와 K' points 에서 valley를 갖게되며(Figure 1), 인가되는 전기장과 자기장에 매우 강력한 valley-selecive interaction을 보인다.
이 물질들에 대한 isolation과 조사는 valleytronics 분야에서 매우 중요한 사건이었다. Figure 2 에 나와있듯이 해당 분야의 출판 histogram을 보면, 2004년에 있었던 그래핀 isolation은 valley physics에 대한 새로운 연구를 촉발시켰다. 그리고 2010년, TMD monolayer에 대한 광학적 특성에 대한 연구가 이루어졌고, 수많은 밸리트로닉스 관련 출판물의 기하급수적 상승을 유발했다. 이후의 논의들은 monolayer 물질이 가지는 근본적인 symmetry가 valley addressability에 있어서 왜 중요한지에 대해 설명한다; 이는 대부분이 이미 발표된 논문(Ref 13-15)을 기반으로 한다.
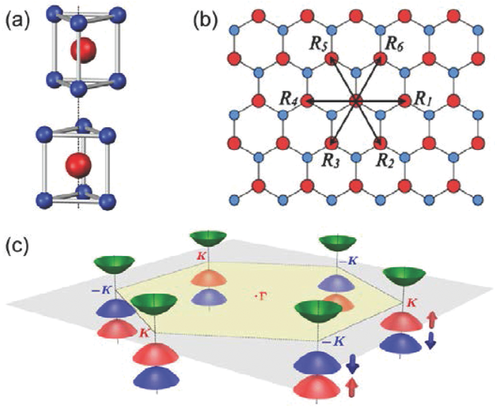
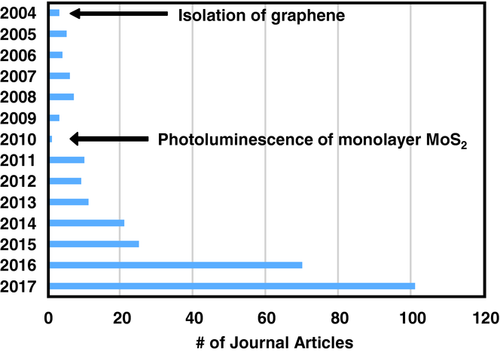
우리는 우선 독자들을 위해 위에서 언급한 symmetry에 대해 자세히 살펴볼 것이며, valley와 관련된 개념들을 명확히 할 것이다. 서로 다른 valley states를 선택적으로 커플링 시키기 위해서, 이 states들을 구분할 실존하는 물리량이 필요한데, 그중 하나가 바로 Berry curvature Ω→ 이다. Berry curvature는 electronic bands의 기하학적 특성을 묘사하는 물리량으로, band topology-related effect를 이해하는데 중심이 되는 물리량이다.
Crystal lattice 속 전자의 움직임을 묘사할 때, 준고전적 운동방정식이 주로 사용된다. 이 묘사에서는 전자가 crystal을 퍼져나가는 Bloch wave로 취급되는데 이때 전자의 평균 속도는 band의 electronic energy의 경사에 비례한다. 여기에는 Lattice의 주기성(by the Bloch form of the electron wavefunction) 뿐만 아니라, 가해지는 전기장, 자기장에 따른 캐리어의 response 또한 고려된다.
하지만, 위에 언급된 contribution 뿐만아니라, 때때로는 무시되는 추가적인 contribution이 존재한다. 이는 electronic band의 Berry curvature에 비례하며, 가해지는 전기장에 수직으로 발생하는 기이한 속도 현상이다.
이는 valley current 뿐만 아니라 보존되는 Berry curvature를 가지는 물질 내에서 발현되는 연관 현상을 가능케 하는 매우 근본적으로 중요한 것이다.
Valley states를 구별하는데 사용할 수 있는 다른 물리량으로는 orbital angular moment가 있다. 직관적으로, 이 물리량은 전자의 wavepacket이 자전하는 것으로 생각할 수 있을 것이며, 특히 전하 캐리어의 spin magnetic moment를 활용할 수 있는 실험과 비슷한 방법으로 valley states를 구별할 수 있기 때문에 유용하다. (예를 들어, 자기장은 spin-up과 spin-down state 사이를 구분 짓는데, 이는 두 state의 magnetic moment가 서로 반대의 값을 가지기 때문이다).
하지만 Berry curvature, orbital angular momentum 그리고 이 두 물리량을 이용해 valley states를 구분하는 능력은, 두 종류의 symmetry가 한 결정 내에 동시에 존재 할 경우 사라지는데, 여기서 말하는 symmetry는 각각 time-reversal symmetry, spatial inversion symmetry이다.
일반적으로, TRS는 시간의 부호 반전에도 계의 symmetry가 있다는 것이며, SIS는 coordinate axes 방향의 반전에도 계가 불변하다는 뜻이다. 이 단순한 symmetry들이 밸리트로닉스에 있어 매우 중요한 결과들을 내놓는다.

Berry curvature, orbital magnetic moment들은 모두 pseudovector로서, spatial inversion에 대해 부호가 변하지 않는다. 이러한 성질들을 갖는 pseudovector들은 그러므로 TRS와 SIS가 동시에 존재할 때는 valley states를 구분하는데 사용하기 적절치 않다, as it would vanish identically.
hexagonal 2D 물질의 K, K' points는 서로가 서로에게 timereversed image이다. 그러므로 일반적으로 time reversal에 대해 odd parity를 가지는 물리량들은 valley states를 구분하는데 있어 좋은 후보군이다. 만약 K와 K' points에서 Berry curvature와 orbital magnetic moment가 동등한 값을 갖지 않는다면, 원칙적으론 전기장과 자기장을 각각 이용해 valley states들을 구분 할 수 있을 것이다. 이는 아래에 보일 것이다.
전자기장내에서 nonvanishing Berry curvature를 가지는 Bloch electrons에 대한 준고전적인 equation of motion은 다음과 같다.
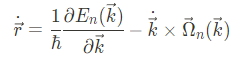

여기서 Ω→은 Bloch function을 이용해 정의될 수 있다.


여기서 ��→은 Berry connection이고 ��은 n번째 에너지 밴드에 있는 Bloch electron wavefunction의 periodic part이다. Berry curvature는 또한 아래와 같이 쓸 수 있다.
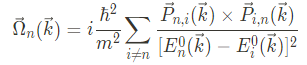
여기서 ��0(�→)는 n번째 밴드의 energy dispersion이며, ��,�(�→)=\bra��|�|��\ket는 velocity operator 행렬의 n행 i열의 성분이다.
Equation of motion이 system이 갖고있는 symmetry에 대해 invariant하다면, TRS에서는 아래의 식이 성립할 것이고,
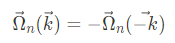
SIS에서는 아래의 식이 성립할 것이다.
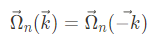
그러므로, SIS만이 깨질 때 valley-contrasting 현상이 발현된다. 다시 말해 아래의 경우에 valley-contrasting 현상이 발현되는 것이다.

Equation of motion을 통해, 2D crystal에 in-plane electric field가 작용할 경우 Berry curvature가 field에 수직한 방향으로 전자의 움직임을 만들어냄을 알 수 있다. 또한 이 전자의 움직임은 valley의 방향에 따라 반대 방향을 향한다.
Broken inversion symmetry는 또한 orbital magnetic moment의 존재를 가능케 한다. n번째 밴드의 electron energy dispersion은 아래와 같이 변한다.

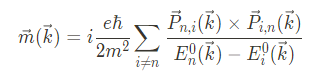
Finite orbital magnetic moment의 존재는 또한 valley 캐리어가 optical circular dichroism을 가질 것을 암시한다. 예를 들어, 이 캐리어들은 좌원편광이냐 우원편광에 대해 illumination 시에 서로 다른 특성을 띈다.
비록 opticla circular dichroism이 TRS가 깨진 system에도 존재하지만, 밸리트로닉스 물질들의 기저 물리는 매우 다르고, dichrosm이 TRS가 유지될때도 존재한다는 점을 명시해야 한다. orbital magnetic moment의 효과중 하나는 valley optical selection rule이다.
구체적인 예시로서, 많은 2D TMDs의 2H phase는 inversion symmetry가 부재하고, 이에 따라 K와 K' valley에서 서로 반대되는 Ω→ ,�을 갖는다. K와 K' points 부근의 band edge에서 �→⋅�→ Hamiltonian은 아래와 같이 주어진다.
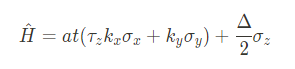
여기서 �는 lattice spacing, �는 nearest-neighbor hopping integral, ��는 valley index로 1, 또는 -1의 값을 가지는 수 이고 �는 Pauli matrix element, 그리고 마지막으로 Δ는 밴드갭이다.
이 경우, conduction band에서의 Berry curvature는 다음과 같이 주어진다.
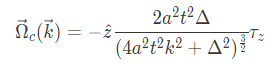
두개의 서로 다른 valley에서 반대의 부호를 가지는 Berry curvature 때문에, in-plane 전기장은 캐리어에 'Valley Hall Effect'(VHE)라는 현상을 유발한다. (Figure3)
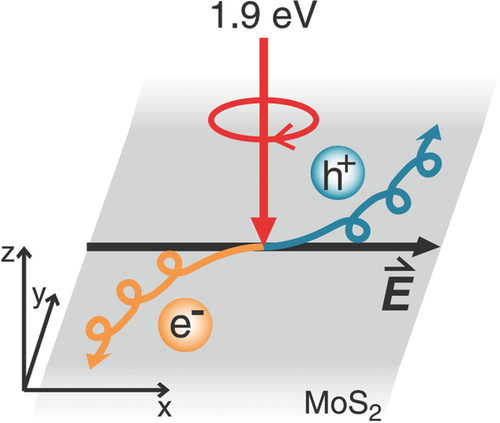
이때 valence band의 Berry curvature와 conducion band의 Berry curvature는 부호는 반대이지만 값은 같다는 점을 알아두자.
Orbital magnetic moment는 valence band와 conduction band에서 아래와 같이 서로 같은 값을 갖는다.
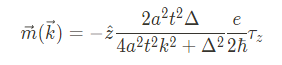
Orbital magnetic moment가 0이 아닌 값을 갖는다는 것은 valley가 상반되는 magnetic moments ( ��=±1)을 갖는다는 것이고, 그러므로 magnetic signature를 통해 valley polarization을 찾을 수 있다. Orbital magnetic moment는 또한 대간천이에 대한 circularly polarized optical selection rule을 발생시킨다.
Berry curvature, orbital magnetic moment, 그리고 optical circular dichroism \vec{\eta}_{\vec{k}는 아래와 같이 연관된다.
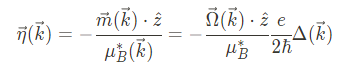
여기서 ��∗과 Δ(�→)는 각각 �→에서의 direct transition energy와 band gap이다.
K와 K' points의 에너지 국소점에서는 ��→=−��가 full selectivity를 가질 것이다. K에서의 transition은 �+인 빛에서만 커플링 될 것이고, K'에서의 transition은 �−인 빛에서만 커플링될 것이다. 이 selectivity는 valley polarization에 대한 optical preparation, control, 그리고 detection을 가능케 한다.
요약하자면, 만약 Berry curvature가 K와 K' points에서 서로 다른 값을 가진다면, 인가되는 전기장에 따라 각 valley에서 서로 다른 행동을 예상할 수 있다. 만약 orbital magnetic moment가 K와 K' points에서 서로 다른 값을 가진다면, 인가되는 자기장에 따라 각 valley에서 서로 다른 행동을 보일 것이다. 이 처럼 K와 K' points에서 상반되는 Ω→와 �→ 값은 두 valley 사이에서 optical circular dichroism을 유발하여 right or left helicity를 가지는 photon에 의한 selective excitation을 가능케 한다. Ω→와 �→ 이 TRS가 유지됨에도 서로 상반되는 값을 가지기 위해서는 물질의 SIS 특성이 부재해야 한다. Though spatial inversion symmetry can be induced in gapped graphene, for example, by biasing the substrate underlying bilayer graphene, monolayer 2D transition metal dichalcogenides meet this requirement without the need to externally introduce a band gap or symmetry breaking, and thus TMDs appear to be the most promising candidates for useful valleytronic applications.
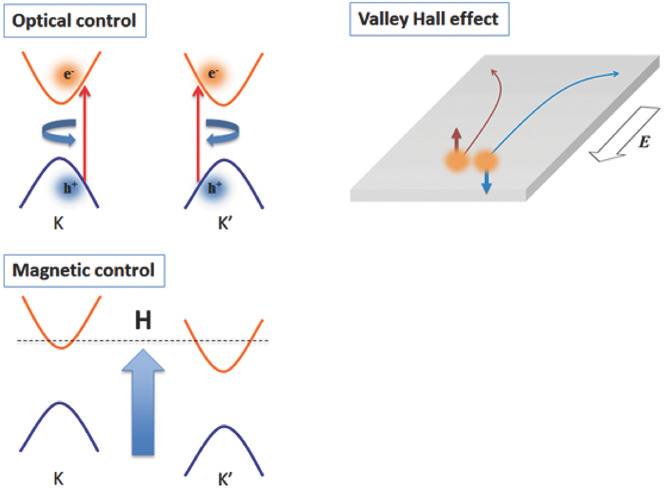
3. Emerging Opportunities and Technical Challenges
The focus of the valleytronics research community thus far has been on material growth, characterization, and valley physics experimentation. There has been limited consideration of practical devices or systems, which would exploit valleytronic properties. Thus it was important to devote time in the Workshop to discussion of the most promising applications for valleytronics and what are the greater technical challenges to realize these applications. Those useful technology implications are presented in this section.
3.1. Quantum Computing
Using TMDs as qubits for quantum computing has some very attractive potential benefits. It may be significantly easier to integrate thousands or millions of valley qubits on a layer of TMD in a simple planar architecture as compared to other modalities such as trapped ions. Because spin–orbit coupling provides energy separation between spin-up and spin-down states, and the valley index provides momentum separation between K and K′ states, each quantum index provides a degree of protection of the other index. So spin protection of valley or valley protection of spin may provide a more favorable gate to coherence time ratio than unprotected qubit candidates. Because valley qubits are interrogated at optical frequencies, single qubits could be addressed through submicrometer waveguides allowing a higher density of qubit packing compared to that for superconducting qubits, which are limited by microwave transmission lines and inductors. Gate operation times may be concurrently faster as well. On the other hand, there is the notable concern that large spin–orbit coupling will increase the interactions between the valley qubit and its environment, thus reducing coherence time. It is also important to note that entanglement between two valley qubits has not yet been demonstrated, and it is possible that the large separation in momentum space between the K and K′ valleys may make entanglement difficult to realize.
5. Valleytronic Material Growth and Device Processing Challenges
5.1. Domain Size and Grain Boundaries
Valleytronic properties are predicated upon the solid-state physics of an ideal single crystal. By contrast, an amorphous film of MoS2 presumably will not exhibit any useful valleytronic effect. In the absence of data to the contrary, we assume that ideal valleytronic effects will be observed only if the entire active area of the device or circuit is composed of a single crystalline domain. Polycrystalline material may exhibit some degree of valleytronic behavior within each small
5.4 Multilayer TMDs
This paper has almost exclusively considered monolayer 2D materials for valleytronics, since the spatial inversion symmetry is restored in bilayers and thus valleytronic properties are lost. However, in the presence of a substrate, an electric field, or a strain field, the inversion symmetry of a bilayer is broken again, and valleytronic behavior is (at least partially) restored. One could try to exploit this effect by applying an electric field across a bilayer material to turn valley currents on and off. For example, in a monolayer of TMD material, the conductivity due to the VHE cannot be easily tuned, and its magnitude is intrinsically linked to the doping level. It is difficult to turn on and off the VHE, as one would want do with a valleytronic switch. In bilayer TMDs, the VHE is absent as inversion symmetry is restored. However, electrostatic gating of bilayer TMDs induces some degree of symmetry breaking, in effect switching between a bilayer and two noninteracting monolayers. The strong dependence of both the magnitude and polarity of the Valley Hall conductivity on the applied field presents a way to electrically tune the VHE in bilayer TMDs, and thus bilayers of TMDs may be useful for selective valleytronic transport.
Bilayers of stacked 2D materials (Figure17) provide an additional rotational degree of freedom to control valleytronic effects as well. The Moiré patterns in stacked TMD bilayers produce periodic potential wells as deep as 160 meV. These may be useful for creating quasi-atomic lattices of particles that can be employed as arrays of devices for memory or logic operations. It is known that exciton-polaritons can condense into a Bose–Einstein condensate (BEC). If the BECs at given lattice points in the Moiré pattern can be made to interact, it may be possible to realize the BEC equivalent of an atom interferometer.
6 Summary and Outlook
Valley degree of freedom의 manipulation과 연관된 새로운 물리는 기념비적인 영향을 끼칠 가능성이 있다. 섹션 3에서는, 밸리트로닉스를 이용한 정보 처리와 광공학적인 요소에 대한 다양한 아이디어들 보였다. 동시에, 저자는 실제 시스템에서 퍼포먼스적 이점을 가지는 밸리트로닉스 디바이스에 대한 개념과 아이디어는 아직 출판되지 않았다고 단언한다. 이것이 밸리트로닉스를 그저 흥미로운 물리현상이 아니라 technology element라 주장할 때 주요한 결점(차이)이다. 사실, valleytronic 특성(valley lifetime, valley coherence time, and valley mean free path)들이 유용한 기능을 수행하기에 치고 넘친다는 점에 대한 설명도 아직 더 필요하다. Valleytronic behavior가 상온에서도 충분히 유지되는지 여부가, 과연 밸리틀닉스가 application 레벨에서도 적합한지에 대한 질문에 답을 해줄 것이다; robust valleytronic behavior at room temperature is still an open question.
It is commonly stated upon the discovery of a new material or physics phenomenon that it will enable new products and technologies. However very few new discoveries actually develop in to real products. To achieve real-world impact the integrated device needs to provide significant performance or power advantages over the existing technology, preferably at similar cost. This is a formidable challenge, and we have attempted to be quite realistic in our assessment of the potential impact of valleytronics. We have identified several areas in which the unique physics of valleytronic materials could meet this challenge.
We need to establish concrete device concepts, improve device processing, understand the structure–process–properties relationships of valleytronic materials, improve single-crystal growth, and demonstrate arbitrary rotations of the valley state around the Bloch sphere, among other things. The required research investment is significant and must be well coordinated. Meeting these challenges will require an interdisciplinary and convergent approach employing experts in materials science, solid state physics, device engineering, and computer science.
https://physics.stackexchange.com/questions/23019/time-reversal-symmetry
Time-reversal symmetry
For a quantum system with time-reversal symmetry, other than the absence of a magnetic field, can we infer anything else about the system?
physics.stackexchange.com
Symmetry arguments for valley physics in graphene with broken inversion
I am trying to understand this paper: http://link.aps.org/doi/10.1103/PhysRevLett.99.236809 (Here is an arXiv version: http://arxiv.org/abs/0709.1274) In the introduction, they mention certain sy...
physics.stackexchange.com
https://physics.stackexchange.com/questions/127531/lack-of-inversion-symmetry-in-crystal
Valley-spin Hall effect
해당 글은 Physics Stack Exchange에 올라온 질문을 번역한 글입니다.
Q)
TMDs에서의 발생하는 valleytronics에 대해 공부를 하고 있는 중입니다. 여러 논문들을 찾아봤는데, 해당 논문들에서는 valleytronics가 그저 time-reversal symmetry 때문에 발생한다고 쓰더군요. 누가 좀 더 쉽게 설명해줄 수 없을까요?
A)
Monolayer TMDs에서는 inversion symmetry가 깨져있습니다. 이에 따라 결과적으로 첫번째 Brillouin zone의 K valley와 K' valley 부근에서 electron의 bands가 non-zero Berry curvature를 갖게되죠.
게다가, 이 화합물은 강력한 SOC를 가지고 있으며 이를 통해 valence bands에서 spin-splitting을 유발합니다(and to a much lesser extent in the CB). TRS는 두개의 valley에서 각각 발생하는 splitting이 반드시 반대가 되어야 한다고 요구합니다. 예를 들어, 한 valley에서는 spin-up bands가 높은 에너지를 가지지만, 반대 valley에서는 spin-down bands가 높은 에너지를 가지는 것처럼요.
이는 결과적으로 spin과 valley의 degree of freedom 끼리의 coupling을 유발하고, 이를 통해 우리는 서로 다른 valley에서의 각기 다른 spin의 carrier를 여기시킬 수 있습니다. 보통 이러한 control은 probing optical fields에서의 frequency, polarization 조절로 이루어지죠. 우리는 이를 valleytronics라 부릅니다.
Also, note that such an optical control of the valley degree of freedom is difficult in graphene due to the absence of strong spin-orbit coupling.
This paper is a good reference: D. Xiao, G.-B. Liu, W. Feng, X. Xu, and W. Yao, "Coupled Spin and Valley Physics in Monolayers of MoS2MoS2 and Other Group-VI Dichalcogenides", Phys. Rev. Lett. 108, 196802 (2012).
'Other Topics' 카테고리의 다른 글
Wavepacket (파속) in Quantum Mechanics (0) | 2023.03.13 |
---|---|
Eigenstates의 확률 진폭의 분포를 나타내는 두가지 방법 (1) | 2023.03.13 |
Spintronics Measurement (1) : Harmonic Hall voltage measurements 를 이용한 스핀궤도토크 구하기 A to Z (1) | 2023.02.27 |
(작성중) 오비트로닉스 (Orbitronics) (0) | 2022.08.29 |
교환 상호작용 (Exchange interaction) (0) | 2021.05.27 |
댓글