참고문헌
[1] FMR-related phenomena in spintronic devices, (Yi Wang et al 2018 J. Phys. D: Appl. Phys. 51 273002, 10.1088/1361-6463/aac7b5)
[2] MEASUREMENTS OF SPIN TORQUES GENERATED BY TOPOLOGICAL INSULATORS AND HEAVY METALS, Alex Mellnik
[3] CHARGE TO SPIN CURRENT EFFICIENCY STUDIED BY SPIN TORQUE FERROMAGNETIC RESONANCE IN VARIOUS METAL HETEROSTRUCTURES,Weifeng Zhang
[4] GENERATION OF SPIN CURRENTS IN FERROMAGNETIC MATERIALS, Joseph Arthur Mittelstaedt
[5] 자성재료와 스핀트로닉스 - 스핀-토크 자기 공명법 측정 이론, 이억재
* 해당 글은 CGS unit을 기본적으로 사용합니다.
1. Introduction
HDD read head 분야에서 스핀 밸브가 설공적으로 적용된 이후, 비휘발성 MRAM과 스핀 논리 소자와 같은 새로운 스핀트로닉스 소자들에 대한 연구가 지속되고 있다. 스핀트로닉스 기반 소자의 활용 측면에서, 주요 목표는 전기적 수단을 통해 더 빠른 동작 속도와 낮은 에너지 소비를 실현하며 자화를 효율적으로 제어하는 것이다. 이를 달성하기 위해서는 자화의 전기적 제어의 효율성을 제고하는 것이 필수적이며, 이러한 필요성에 따라 전류를 주입했을 때 발생하는 자화 동역학을 기반으로 하는 다양한 측정 기술들이 개발됐다. 자화 동역학에 대한 연구는 20세기 초중반의 FMR의 개발로 시작됐다. 원래 FMR은 외부 자기장이 가해질 때 자성박막에서 발생하는 자화 동역학을 연구하기 위해 사용됐다. 그러나 1996년 스핀-전달 토크(spin-transfer torque, STT)가 발견되고 현대 나노 공정 기술이 발전하면서 소자의 크기가 기하급수적으로 줄어들게 됐고, 이러한 nanomagnet을 스핀 편극(polarized) 전류를 주입하여 전기적으로 제어하려는 연구가 큰 관심을 받게 됐다. 2000년 이후, STT 기반 자화 반전이 GMR 나노필러와 나노 사이즈의 MTJ 소자에서 실험적으로 증명됐다.
2004년, GaAs라는 단일 반도체에서 스핀 홀 효과(spin Hall effect, SHE)에 의해 직접적인 전하전류-스핀류 변환이 실제로 관측됐다. 이 발견 이후, 비자성체에서 순수 스핀류(pure spin current) 생성에 대한 주제가 스핀트로닉스 분야에서 큰 관심을 끌기 시작했다. 또한, 자화가 순수 스핀류만으로도 스위칭되거나 세차운동 모드에 들어갈 수 있음이 증명됐다. 비자성체에서 순수 스핀류의 생성은 스핀-궤도 결합(spin-orbit coupling, SOC)에 의해 이루어지며, 이 과정은 SOC가 SHE를 통해 순수 스핀류를 발생시키고, 이 전류가 인접한 강자성체층에 토크를 발생시키는 메커니즘으로 이루어진다. 이때 발생하는 토크를 스핀-궤도 토크(spin-orbit torque, SOT)라고 하며, SOT 방식에서 높은 효율의 자성 제어를 달성하기 위한 주요 과제는 높은 전하전류-스핀류 interconversion 효율(spin Hall angle 또는 spin-orbit torque efficiency)을 가지는 물질을 찾는 것이다. Spin Hall angle을 구하는 측정법은 다양하다. 그 중에서, 우리가 오늘 학습하고자 하는 것은 강자성공명(Ferromagnetic Resonance) 기법이다. 이제 아래서 이에 대해 하나씩 살펴보고자 한다.
2. Ferromagnetic Resonance
2.1. LLG equation
Macrospin approximation을 적용했을때, 유효자기장이 걸려있는 계 내의 강자성체 속 자화 벡터 $\vec{m}$에 대한 동역학은 Landau–Lifshitz–Gilebert (LLG) 방정식을 통해 아래와 같이 기술할 수 있다.
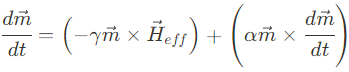
여기서 $\gamma$는 gyromagnetic ratio, $\mu_{0}$는 vacuum permeability, 그리고 $\alpha$는 Gilbert damping parameter이다. 위에 기술된 LLG 방정식의 우항에는 두개의 토크항이 존재한다. 이중 첫번째는 유효자기장 방향을 중심 축으로 하여 자화가 세차운동을 하는 것을 나타내는 항이고, 두번째는 damping (energy dissipation) 항으로 자화가 유효자기장 방향에 평행하게끔 만든다. Gilbert damping parameter $\alpha$는 자화 $\vec{m}$이 얼마나 빠르게 유효자기장 방향으로 향하는지를 알려준다. 이제 스핀-분극 전류(스핀 방향은 $\hat{\sigma}$)가 자화 $\vec{m}$과 상호작용하는 경우를 생각해본다. 이 경우, LLG 방정식은 아래와 같이 수정된다. 또한 아래의 식을 Landau–Lifshitz–Gilebert–Slonczewsk(LLGS) 방정식이라 부른다.

여기서 $\vec{\tau}_{_{DL}}$은 damping-like torque, $\vec{\tau}_{_{FL}}$은 field-like torque이며 아래와 같이 쓸 수 있다.

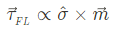
위 식에서 알 수 있듯이, 자화 $\vec{m}$이 xy-plane에 있을 경우 DL torque은 in-plane 방향이며, FL torque는 out-of plane
2.2. Ferromagnetic resonance (FMR)
FMR 측정의 가장 기본적인 방식은 cavity, 또는 인접한 waveguide에서 진동하는 자기장을 이용해 강자성체를 여기시키는 것이다. 이 FMR 현상은 자성 물질의 특성뿐만 아니라 자성 박막에 가해지는 토크를 정확하게 관측할 수 있게 해준다. 이 과정에서, 강자성체의 magnetic order는 일정한 외부 자기장이 가해짐에 따라 perturbation 되어 AC excitation 한다. 여기서 외부 자기장은 자화의 평형 방향을 설정하고, 자화가 공명하는 축으로 작용한다. AC excitation은 자화의 oscillation을 유발하며, oscillation의 진폭과 형태를 결정하는 주요 요소로 작용한다. AC excitation에 대한 oscillation의 민감도, 그리고 이에 대한 세부 사항들을 FMR이 측정함으로써, FMR은 강자성 박막에 미치는 영향을 분석하고 스핀류를 측정하는 주요 방법으로써 자리잡았다. FMR의 정량적 이해를 위해, 우리는 위에서 배운 LLGS 방정식을 도입해야 한다. 가장 일반적인 케이스로 DC term, AC term, 그리고 Gilbert term으로 나타내면

정확한 AC torque는 측정 타입에 따라 달라질 것이다. 하지만 DC torque는 일반적으로 eq. 1에서의 유효자기장 항과 같은 형식을 가진다. 여기서 추가로 말하자면, 이 형식은 system의 magnetic free energy로부터 유도된다. 만약 우리가 free energy function $F(\vec{m})$을 정확하게 알고 있다면, DC torque는 아래와 같이 쓸 수 있다.

eq. 6으로부터, 이미 우리는 강자성체 물질의 일반적인 동역학에 대한 결과를 어느정도 볼 수 있다. AC torque가 없는 경우, DC torque는 자화를 $\vec{H}_{eff}$를 중심으로 세차운동하게 만들것이며, 이때 이 세차운동의 주파수는 effective field의 세기에 비례한다. Damping 항은 자화를 effective field의 방향으로 밀어낼 것이며, 이에 반대 방향으로 작용하는 driving torque가 부재한다면 자화와 effective field를 align 시켜 $\vec{\tau}_{_{DC}}$가 0이 되게끔 할 것이다. 그러므로, 우리가 일정한 oscillation을 원한다면, 시스템에 지속적으로 에너지를 주입시켜 damping에 대한 반대 효과를 만들어내야 한다. 정량적 묘사를 이제 시작해본다. 우리는 우선 free energy $F(\vec{m})$과 AC torque $\vec{\tau}_{_{AC}}$에 대해 기술해야 한다.
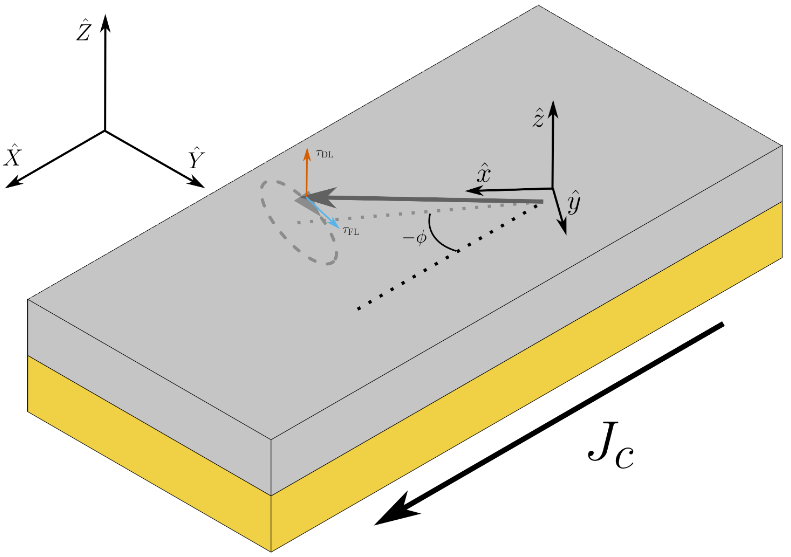
우선 Free energy를 보면, 우리는 박막에서의 가장 기본적인 식을 사용할 것이며, 아래와 같이 나타낼 수 있다.

이 경우, $\vec{\tau}_{_{DC}}$는 다음과 같이 나타낼 수 있다.

AC torque는 매우 다양한 형태로 나타날 수 있으며, 그러므로 특정 FMR 측정 종류에 맞게 바꿔가며 적용을 해야한다. 이는 나중에 다시 기술하도록 하겠다.
시작하기에 앞서, 몇가지 가정을 통해 계산을 용이하게 하고자 한다.
- 우선 첫번째로, 우리는 해당 샘플이 $M_{eff} > 0$을 만족하는 in-plane 자화를 가지는 샘플임을 가정한다. 이에 더해 in-plane anisotropy가 매우 작아 자화의 equilibrium orientation이 거의 applied field와 같음을 가정할 것이다.
- 두번째로 oscillation이 매우 작음을 가정한다, 다시 말해 Taylor expansion을 통해 magnetization oscillation amplitude에 linear 한 term 만을 가져갈 것이다.
이 두가지 가정을 통해, 우리는 Fig.1 과 같은 좌표계를 정의한다. 여기서 $\hat{x}$ 축은 magnetization equilibrium orientation의 방향이며, 우리의 가정을 돌이켜 보면, 이 방향은 외부자기장의 방향이 된다. 해당 좌표계에서 우리는 자화와 외부자기장을 아래와 같이 쓸 수 있다.


eq. 9와 eq. 10을 이용하면, $\vec{\tau}_{_{DC}}$는 아래와 같이 나타낼 수 있다.

여기서 $\omega_y, \omega_z$는 각각 y, z축 방향의 natural frequency로 다음과 같이 나타낼 수 있으며, 추후 분석에 있어 유용한 값이 된다.


만약 시스템 속 에너지 중 더 복잡한 표현이 있는 경우, 해당 단계에서 그 표현식을 적용해야 하며 natural frequency에 변화가 반영 되어야 한다.
LLGS 방정식의 damping portion은 아래의 ansatz를 이용해 단순화 시킬 수 있다.

이 경우, AC torque는 아래와 같은 항을 가지며

이를 통해 자화 $\vec{m}$이 phase difference를 수반하는 것을 허용한다. 또한 아래와 같은 표현도 가능해진다.
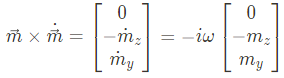
마지막 파트는 AC torque일 것이다. 앞에서 얘기 했듯이 이 항은 가능한 '일반적인 항'으로 나둬야 한다. 우리가 현재 단계에서 이 항에 대해 이야기 할 것은, 우리는 $\hat{x}$ 방향으로는 어떠한 동역학도 일어날 것을 기대하지 않기 때문에, 우리는 $\vec{\tau}_{_{AC}}$를 아래와 같이 기술할 수 있다는 것이다.
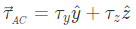
위의 단순화 가정을 통해, 우리는 LLGS 방정식을 풀 준비가 됐다.
여기서, 지금까지 우리가 적어놓은 항들에 대해 다시 한번 살펴보고자 한다.
- 첫번째로, 우리의 smallness approximation은 magnetization equilibrium orientation에 동역학이 발생하지 않음을 의미하며, 이는 3D 문제를 2D 문제로 바꿀 수 있음을 뜻한다.
- 두번째로, 모든 것이 자화에 linear 하기 때문에, 우리는 이를 행렬(또는 텐서) 형태로 쓸 수 있다.

여기서 $\vec{\tau}$는 시스템에 다이나믹스가 있게끔 하는 외부 토크이며, 우리의 경우 이를 $\tau_{_{AC}}$라 한다. Ansatz와 eq. 5의 식을 가져오면, 우리는 suscptibility matrix를 구할 수 있다.


Susceptibility 텐서는 아래와 같이 정리된다.

여기서 damping이 매우 작음을 가정하고 $\alpha$의 제곱항을 날려버리면 아래와 같이 전개가 가능하다.

여기서 resonance frequency ($\omega_0^2 = \omega_y \omega_z$), 그리고 natural frequency ($ \omega^+=\omega_y+\omega_z$)라는 물리량을 각각 정의 한 뒤, 분모를 유리화 시키면 최종적으로 아래와 같은 식을 얻을 수 있다.

위의 최종 식을 보면, $\omega_0^2$를 중심으로 하며 linewidth가 $\omega\omega^+ \alpha$인 Lorentzian의 형태를 가짐을 알 수 있다. 또한 imaginary part는 일반적인 symmetric Lorentzian을, 그리고 real part는 antisymmetric Lorenzian을 나타냄을 알 수 있다.
이 항들이 추후 중요해지는데, 이는 측정에서 나오는 여러 요소들을 분리시켜주고 공명의 퀄리티를 측정하게 끔 해주기 때문이다.
우리가 가정한 시나리오에 대한 계산은 끝이 났지만, 해야 할 일이 더 남아있다. 우선, 우리의 현재 표현은 주파수 기반의 작성법이다. 하지만 일반적으로 H sweep이 freq sweep보다 더 쉽고, 더욱이 우리는 H에 대한 항으로 이루어진 표현을 더 선호한다. Field dependence의 주된 근원은 외부 장에 따른 resonant frequency $\omega_0$의 변화로 부터 온다, 그러므로 우리는 이를 자기장에 대해서 1차항까지 전개하여 변수를 바꿀 것이다.
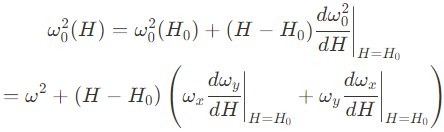
정리하면

위의 전개 과정에서 나오는 관계식$\omega^2=\omega^2_0(H_0)$은 정의에서부터 온다: 우리가 외부장을 sweep할 때, 우리는 상수의 driving frequency $\omega$를 가지며, field strength를 바꿔감에 따라 resonant frequency $\omega_0$가 변화한다. Field space에서의 resonance를 일으키는 field를 $H_0$로 우리가 정의하기 때문에, $\omega_0(H_0)$는 resonance가 "발생해야 하는" 주파수가 되며, driving frequency $\omega$와 같게 된다.
위의 식에서 나오는 $\omega_x$, $\omega_y$, $\omega^+x$ 모두 field dependence를 가져야 함을 알 수 있을 것이고 이를 식으로 나타내야 한다. 하지만, 대부분의 경우 동역학은 resonant field 근처에서 일어나며, 그렇기에 $H_0$에서의 $\omega_x$, $\omega_y$, $\omega^+x$의 값을 대입하는 것 역시 나쁘지 않은 approximation이다.
이제 우리는 eq. 23의 susceptibility를 field coordinates에서 작성할 것이다. 이를 통해 우리는 실험과 계산의 직접적인 연결성을 더욱 획득할 수 있다.

식을 조금 정리하면

여기서 우리는 다음의 물리량을 정의한다. 이 물리량은 Lorentzian lineshape의 linewidth를 나타낸다. 또한 이때 Lorentzian의 FWHM은 $2\Delta$이다.

이를 도입하면 Susceptiblity에 대한 식은 아래와 같이 나타낼 수 있다.

이 형태의 susceptibility 공식이 대부분의 측정에서 활용하기에 편리하다.
일반적으로는 FMR 측정을 진행 할 때, 특정 공명의 정보에 집중하는 편은 아니다. 대신에 oscilalting external field의 frequency를 바꿔줌에 따라 resonant field와 linewidth가 어떻게 변화하는지에 대해 초점을 맞춘다.
측정은 field sweep으로 이루어지며, 이때 field points는 매우 겹겹이 있어 적은 set의 frequency $\omega$에 대해서도 resonance lineshape가 매우 쉽게 resolved 되며 resonance position $B_0$와 linewidth $\Delta$ 역시 쉽게 피팅 된다. 해당 측정은 다음의 두개의 물리량을 구하기 위해 사용된다
- The effective magnetization
- Damping constant
위에서 정의했듯이, resonance frequency의 제곱은 y, z축 방향의 natural frequency의 곱으로 나타나며, natural frequency는 matrix form으로부터 구했으므로, 이를 정리하면 아래와 같이 쓸 수 있다.

이를 $H_0$에 대해 근의 공식을 이용해 정리하면 아래와 같다.
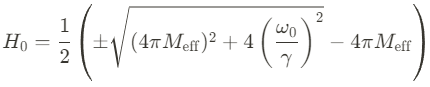
위의 방정식이 바로 박막에서의 Kittel relation 중 가장 간단한 형태이다. 우리는 이 식을 이용하여 resonant field를 주파수의 함수로 피팅하여 effective mangetization을 결정할 수 있다.
Damping constant $\alpha$를 찾기 위해서, 우리는 그저 우리가 linewidth $\Delta$를 $\omega \alpha / \gamma$의 값으로 정의 했다는 것만 기억하면 되며, 단순히 linewidth를 주파수의 함수로 피팅만 하면 damping constant $\alpha$를 구하는데 충분할 것이다. 하지만, 실제 결정 내 불완전성 때문에, 주파수에 independent한 linewidth의 broadening이 유발될 수 있으며, 아래와 같이 상수 linewidth를 더함으로써 반영할 수 있다.
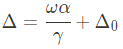
However, there are some limits of FMR technique. The signal is from the absorption of the microwave power, so the input power has to be large. And the sample needs to be large enough to observe the difference.
3. Spin-Torque FMR
이제, 교류자기장을 인가하여 resonance를 일으키는게 아니라 교류 전류를 이용하여 강자성체의 세차운동을 유발하는 ST-FMR을 알아보고자 한다. 해당 연구 방법은 spin Hall scheme의 Pt/NiFe(Py) bilayer 에서 처음으로 사용되어 charge-to-spin conversion efficiency (spin Hall angle)를 평가하는 효과적인 방법임이 증명됐다. ST-FMR의 경우, 자성 레이어에서의 강자성 공명은 FM에 인접한 스핀 소스 레이어에 흐는 전류가 만들어내는 AC spin torque에 의해 유발되며, 뿐만 아니라 소스 레이어에 흐르는 전류가 발생시키는 Oersted torque도 공명에 가담한다. Magnetoresistance 효과들에 의해 발생한 저항들은 당연하게도 magnetization oscillation과 연관될 것이고, 자연스럽게 저항도 oscillation을 할 것이다. 이때 이 resistance oscillation과 인가되는 AC전류의 mixing에서부터 DC voltage가 만들어지고 해당 값을 이용하여 우리는 oscillation의 정도를 관측할 수 있다.
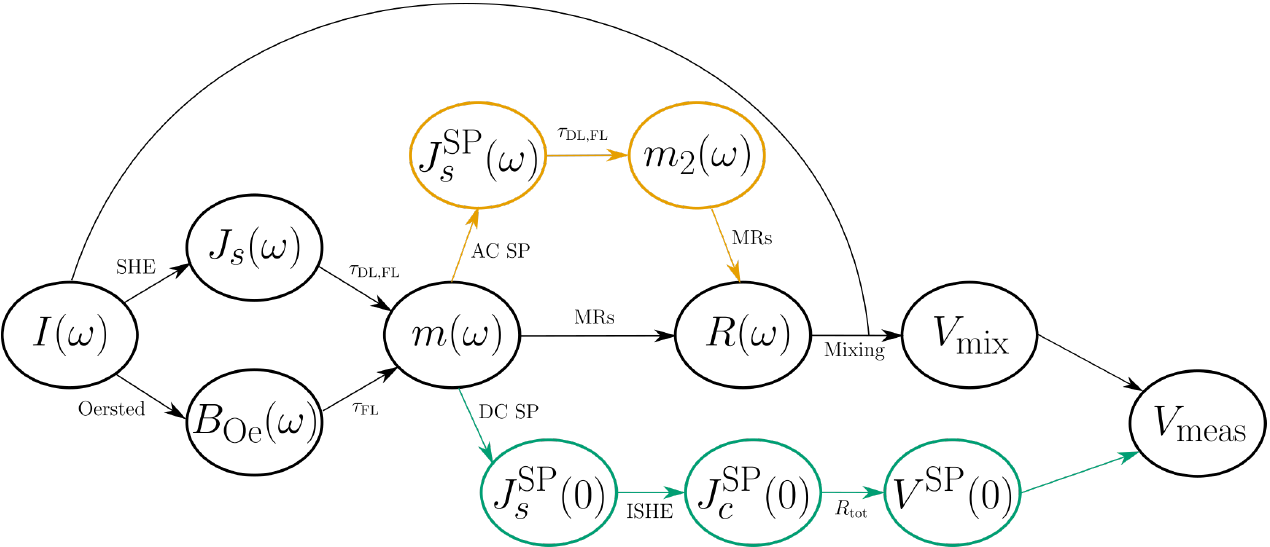
Fig. 2 에서 검은선으로 나타나 있는 것은 AC current가 torque를 유발하고 결과적으로 자화가 oscillation 하게끔 만드는 주된 경로이며, resistance oscillation이 AC current와 mixing 후 DC mixing voltage가 만들어지는 것을 알 수 있다. 하단부의 청록색 경로는 DC pumped spin current에 이어 ISHE가 발생하여 또 다른 DC voltage가 만들어짐을 보여준다. 상단부 노란색 경로는 AC pumped spin current가 두번째 magnetic layer(존재 할 수도 있고, 안할 수도 있음)에 oscillation을 유발하여 추가적인 저항의 oscillation을 유발하는 과정을 보여준다. 그림에서 알 수 있듯이 ST-FMR 측정에서부터 나온 여러 신호에 대해 이해하기 위해 우리가 알아야 할 몇가지 요소들이 있으며, 이를 아래에서 살펴볼 것이다.
1. 첫번째로는 자화의 oscillation을 찾는 것이다. 우리는 이미 susceptibility의 정보를 갖고 있기 때문에, 우리가 필요로 하는 것은 단지 어떤 torque가 excitation을 유발하는지 아는 것이다. 지금은, 인가된 전류와 자화 사이의 각도가 변화할 때 토크의 effective strength가 어떻게 변하는지에 집중할 것이다. 스핀류의 spin polarization $\hat{\sigma}$가 주어지면, 토크의 방향과 세기가 전류와 자화 m 사이의 상대적인 방향에 따라 어떻게 변화하는지 알 수 있다. (우리는 전류의 방향을 언제나 $\hat{X}$ 방향으로 가정 할 것이며, Fig. 1에 나와있는 coordinate system을 이용할 것이다).
Spin Hall effect($\hat{\sigma}=\hat{Y}$)에 의해 만들어지는 DL torque 를 예로 들면, torque의 방향은 아래와 같이 주어진다.

만약 자화가 전류 방향에 대해 $\phi$만큼의 각도를 가지고 있다고 가정하면, 자화에 가해지는 토크의 값은 아래와 같이 정사영 된 벡터값을 나타낼 것이다.

위와 같이 $\hat{\sigma}=\hat{Y}$을 가지는 경우에 field-like torque를 보면, (일반적으로 Oersted torque를 예로 들자) torque는 다음과 같은 항을 갖는다.

우리는 임의의 방향의 spin polarizaion에 대해서도 magnetization angle dependence를 아래 Table3.1과 같이 구할 수 있다. 이 angle dependence는 여러 torque들로부터 발생하는 신호 contribution에 대해 역할을 구분시킬 수 있게 만든다.
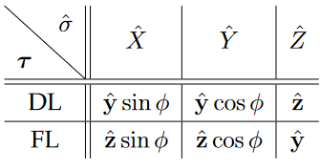
2. 두번째 요소는 전류와 저항의 mixing을 통해 발생하는 DC voltage를 이해하는 것이다. ST-FMR 실험에서, 우리는 AC전류 $I(t)=I*e^{-i \omega t}$ (이때 $I$는 purely real이다)를 인가하며, 이는 자화의 oscillation을 유발한다. 결과적으로 저항의 oscillation을 같은 주파수(phase는 변화할 수 있다.)로 야기시킨다.

이 상황에서 옴의 법칙을 이용하면,

이 옴의 법칙에 위에서 언급한 AC전류와 oscillating 저항을 대입하면,
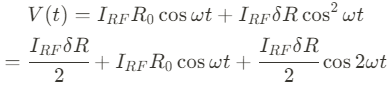
위의 식으로 부터, 우리는 분명하게 주파수에 독립적인 DC component가 나옴을 볼 수 있다. 이 전압 decomposition은 ST-FMR이 상대적으로 쉬운 기술인 이유이기도 하다. 우리는 그저 자화의 RF oscillation 정보를 얻기 위해 DC voltage만 측정하면 되니 말이다.
3. ST-FMR을 분석하는데 필요한 마지막 요소는 우리가 위에서 논의한 저항의 oscillation을 만들어주는 magnetoresistance이다. 연구하는 시스템의 종류에 따라, 그리고 측정 geometry에 따라 여러 종류의 서로 다른 magnetoresistacne들이 영향을 끼친다. 전형적인 강자성체/비자성체 bilayer 시스템에서는 AMR, AHE, PHE, 그리고 SMR등을 고려할 수 있을 것이다. 이러한 저항들은 인가되는 전류의 수직 또는 평행한 방향을 기준으로 했을 때 자화 $\vec{m}$에 대한 dependence와 유도되는 전압의 방향에 따라 구분된다. 이 magnetoreistance들은 아래 Table3.2에 요약 되어있다.
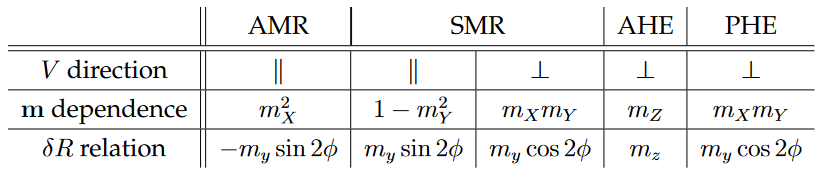
우리가 위에서 봤듯이, 이 신호들은 저항의 oscillation에만 dependent하며, 그러므로 우리는 이것들을 자화의 평형점 $\vec{m}_0$에서 Taylor expansion을 취해 magnetization oscillation을 고려함으로써 이 둘을 연결 시킬 수 있다.
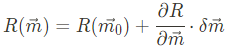
다시 말해, ST-FMR 신호는 사실 MR의 미분값에 의존하는 것이다. 어떻게 작동하는지 보기 위해서, 우리는 AMR을 예로 들고자 한다. 자화의 XY 평면 내의 각을 azimuthal angle $\phi$라 하고, Z축으로 부터의 polar angle을 $\theta$라 할 때, AMR은 아래와 같다.

이를 위의 eq. 39 식에 집어 넣으면 (φ와 θ는 평형 자화 각도에서 평가되며, δφ와 δθ는 각각의 방향에서 발생하는 진동을 나타낸다.)

Fig. 1의 시스템을 생각했을 때, 우리가 첫번째로 알 수 있는 것은 in-plane 방향의 oscillation $m_y$가 정확히 $\delta \phi$와 일치하다는 것이며, out-of-plane oscilaltion $m_z$는 $-\delta \theta$와 거의 유사하다는 것이다. 두번째로, 우리는 in-plane magnetized sample을 고려하고 있으므로 $\theta = \pi/2$로 볼 수 있으며, 이를 통해 식을 아래와 같이 단순화 시킬 수 있다.

우리는 거의 동일한 방식을 이용하여 다른 magnetoresistance에 대해 resistance oscillation과 magnetic oscillation 사이의 관계를 찾을 수 있으며, 이는 Table 3.2. 맨 아랫줄에 나와있다. 이제 우리는 ST-FMR 측정으로부터 얻어진 신호를 분석하기 위한 모든 필요 요소들을 알았다. Eq 3.11.에 나와있는 susceptibility로 부터, 우리는 다음을 구할 수 있다.


위 식에서 우리는 damping의 high order term인 $\delta 2 \alpha$ factor는 모두 소거하였다. longitudinal ST-FMR의 경우, AMR이 primary magnetoresistance이므로, 지금은 이 MR만 고려하고자 한다. Resistance oscillation 대한 표현과 이것이 전압과 어떻게 관련되는지로부터, 우리는 결과 전압의 DC 부분이 다음과 같은 형식을 취한다는 것을 알게 되었다.

위의 식에서 우리는 $\hat{Y}$방향의 polarization을 spin들로부터 발생하는 damping-like torque와 $\hat{Y}$축 방향의 external field로 부터 나오는 Oersted torque를 다음과 같이 가정하였다.
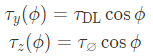
또한 여기에서는, 다른 소스로부터의 field-like torque는 없음을 가정하였지만, 이는 언제나 그렇지는 않다는 것을 알 것이다. Pai et al의 연구에서도 나와있지만, 계면에서 발생하는 SOI에 의한 field-like torque역시 존재할 수 있으며, 이때 이 FL torque를 만드는 spin의 방향은 Oersted field와 같을 것이다. 하지만 저자가 언급 했듯이, 두께별로 토크를 측정함에 따라 이 contribution은 서로 떼어내질 수 있다. 하지만 우리는 단순한 경우만 가정할 것이며, 이때는 Oersted field에 의한 FL torque외에는 고려하지 않을 것이다.
측정되는 전압을 분석할 때, 우리는 V(B) curve를 symmetric Lorentzian

그리고 anti-symmetric Loretzian

의 합으로 fitting한다.
Symmetric component는 외부자기장이 resonance field $H_{0}$일때 최대값을 가지며, 반대로 antisymmetric component는 resonance field $H_{0}$ 일 때 0의 값을 가지며 dispersive curve를 갖는다. 두 항 모두 한 자기장에 대하여 같은 linewidth $\Delta H$를 가진다. 위의 $V_{\mathrm{mix}}$ 식에서 위의 $L_S$, $L_A$ 의 계수를 끄집어내면


위 식을 보면 알 수 있들이, symmetric과 anti-symmetric 대부분의 factor들이 대부분 일치함을 알 수 있다. 이는 다시 말해 ST-FMR 분석 방법에서 spin source layer로부터 spin Hall angle을 구하는 방법에는 $V_{S}$와 $V_{A}$의 비율을 구함이 중요하다는 것을 알 수 있다. 위 식을 $tau$에 관한 식으로 정리하면 아래와 같다.

여기서 우리는 $\omega = \omega_0$가 공명 주파수라는 사실을 사용했으며, $\omega_0 = \sqrt{\omega_y \omega_z}$는 eq. 23 으로 부터 도출되었고, $\omega_y$와 $\omega_z$의 정의는 eq. 12, eq. 13에서 온 것이다. Oersted torque의 경우, 샘플의 geometry에 비해 torque가 작용하는 지점은 계면이므로, 무한 평면상에서 current에 의한 torque로 봐도 무방하다. 이 가정을 곁들이면 아래와 같이 $\tau_{\varnothing}$을 구할 수 있다.
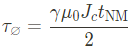
Damping-like torque의 경우, the correct expression relating this to the spin Hall efficiency is (해당 내용은 Physical Review Letters, 106(3):036601, jan 2011 에 나와 있습니다.)
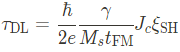
위 두개의 torque식을 저 위에 있는 식에 대입하고 efficiency에 대해 구하면, charge-to-spin conversion efficiency (spin Hall angle)이 나오며 식은 아래와 같다.

위 식에서 우항에 있는 모든 parameter들은 직간접적으로 ST-FMR로 측정이 되거나 다른 측정 수단으로 얻을 수 있는 것들이다. 이 ST-FMR의 "self-calibrated" 특성은 이 측정방법이 "효율적"인 이유 중 하나이다. 우리는 이 방법이 self-calibrated됐다고 하는데, 이는 우리가 RF current가 흐르는 device에서 실제 무언가를 측정할 필요가 없기 때문이다.(실제로 어렵기도 하다). 심지어 magnetoresistance 마저 직접적으로 측정하지 않는다. 이 모든 물리량들은 그저 symmetric voltage와 antisymmetry voltage에 녹아있다. 또한 측정에 영향을 끼칠 수 있는 parasitic term이 많지 않은 것 역시 장점 중 하나이다.
$Parasitic term 중 M_{eff}$, g값은 Kittel formula를 이용하여 추출할 수 있다. $M_S$ 값은 VSM찍어서.. (un-patterend film으로)
이 방법은 FM magnetization에 가해지는 torque가 오직 Pt layer에 흐르는 $J_{\mathrm{C}}$에 의한 것이라고 self-calibration 된 상태에서 계산 되는 것으로, ST-FMR소자의 정확한 $I_{\mathrm{RF}}$값이나 $H_{\mathrm{RF}}$값을 모르고도 spin Hall angle을 구할 수 있다. 이 방법은 $V_{\mathrm{A}}$가 오직 $H_{\mathrm{RF}}$에 영향을 받는다는 가정 하에서도 $\theta_{\mathrm{S}}$의 값을 쉽게 구할 수 있게 해준다. 다른 말로, 이 방법은 계면 효과(예를 들어 Rashba effect)등에 의해 field-like torque $\tau_{\mathrm{FL}}$이 무시하지 못할 정도로 큰 경우에 잘못된 $\theta_{\mathrm{S}}$ 값을 도출 할 수 있다는 말이 된다. 왜냐하면 $\tau_{FL}$은 $H_\mathrm{RF}$와 비슷하게 antisymmetric Lorentzian line shape를 만들 수 있기 때문이며, 이로 인해 $\theta_\mathrm{S}$ 값이 과대, 혹은 과소포장 될 수 있다. 그러므로, 위의 방법을 사용 하기 전에는 반드시 field-like torque가 negligible 한지 아닌지 확인해야 한다. 또한 이 경우에는 Symmetric term의 V값만 이용하여 spin Hall angle을 구하기도 한다.
_______________________________________________________________________________________
V_{S}$만을 이용하는 경우의 damping-like torque $\tau_{DL}$과 이와 연관된 in-plane SOT efficiency $\theta_{_S}$는 아래와 같이 구할 수 있다. 약간 다른 방법으로 위 식을 다시금 유도해보면,
다시 처음으로 돌아가 저항이 angle에 dependent 한 경우의 Taylor expansion을 쓰면, .



이때 $\cfrac{dR}{d\theta_{_H}}$는 $\theta_{_H}$에서의 angular dependent magnetoresistance이며, $\Delta$는 ST-FMR 신호의 frequency domain에서의 lienwidth이다. $E$는 ST-FMR 소자를 가로지르는 microwave electric field이며, $\sigma_{_S}$와 $\sigma$는 각각 HM layer에서의 spin Hall conductivity와 longitudinal charge conductivity이다.
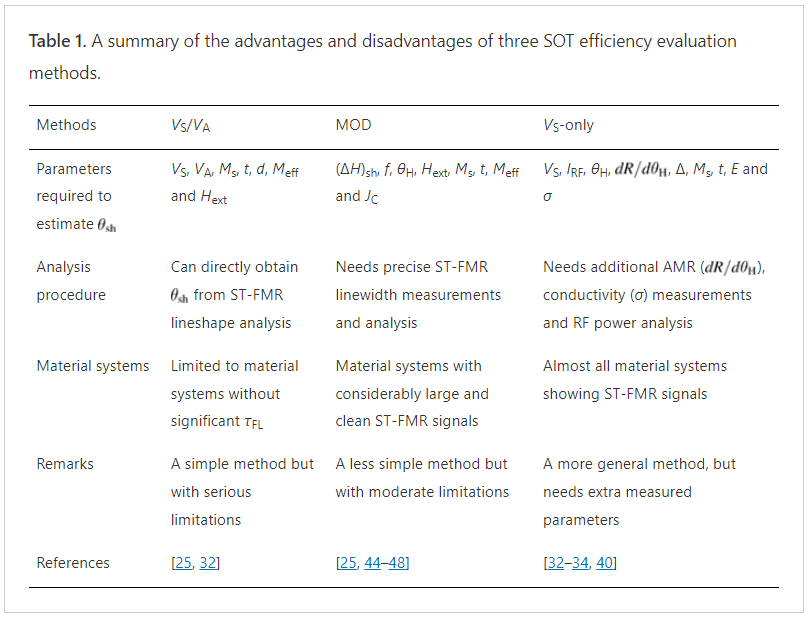
_______________________________________________________________________________________
4. Experimental Details
4.1. Lock-in Amplifier
ST-FMR signal은 rectified DC voltage로서 DC voltmeter를 이용하여 직접적으로 측정이 가능하다. 아래와 같이 Bias Tee의 축전기 부분에 $I_{RF}$를, 코일 부분에 detector를 이어주면 된다. 일반적인 ST-FMR 셋업에서는 앞에서 말한 DC voltmeter가 효과적으로 사용된다. 예를 들어 Pt/Py 처럼 ST-FMR 신호가 $\mu V$대로 상당한 진폭을 가진 경우에는 매우 효과적이다. 하지만 노이즈 레벨이 큰 값을 가지는 경우에는 무시 못할 영향을 끼친다. 특히 100 nV까지 올라가는 경우도 있어 이 때는 $\mu V$대의 ST-FMR 신호를 가지는 물질 조합 연구에 있어 signal-to-noise 비율을 상당히 감소시킨다. 노이즈를 감소시켜 signal-to-noise 비율을 상승시키기 위해, 더욱 정밀한 ST-FMR 셋업이 개발됏으며, 여기서 DC voltmeter는 lock-in-amplifier로 교체됐다. 이는 아래 그림과 같다.
$I_{RF}$는 저 주파수 사인파(modulating signal)에 의해 modulation되는 amplitude이며, Fig 3.(b)에 나와있다. 저주파수 modulating signal은 lock-in amplifier의 reference port에 입력되는 reference signal의 역할을 하며 그러므로 trigger로 작동한다. Modulated $I_{RF}$는 ST-FMR 소자에 주입되며 ST-FMR 신호를 발생시킨다. 이 경우 ST-FMR 신호는 DC 대신에 저주파수의 voltage이며, 이는 위에서 처럼 $I_\mathrm{RF}$의 amplitude가 modulated 되서이다. 저주파수 ST-FMR 신호는 lock-in amplifer에서 phase locking technique를 이용해 관측될 수 있다. 이를 통해 noise level은 10nV 이하로 줄어들며, 기존의 ST-FMR 셋업과 비교했을 때 더 나은 signal-to-noise ratio를 제공한다.
4.2. CPW Design
RF current를 주입할 때 발생하는 두가지 대표적인 현상은 RF power loss와 reflection이다. 이를 최소화하기 위해서는 ST-FMR 소자의 impedance ($Z_m$)가 $50 \Omega$ 정도의 값을 가지는 것이 바람직하다. 첫번째로, CPW는 impedance matching을 고려해서 디자인 되어야 한다. 전형적인 CPW의 예는 Fig. 4(a)에 나와있다. 이때 소스 패드의 너비(s)와 그라운드 패드와 소스 패드의 사이 거리(w)를 조절 함으로써 impedance 특성이 변화한다.
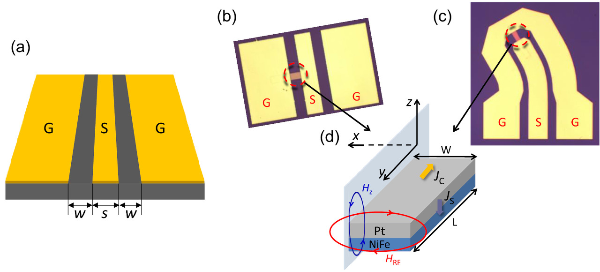
더욱이, HM/FM bilayer current channel 또한 impedance match가 잘 이뤄져야 하며, 이 과정은 채널의 길이(L), 너비(W) 등을 바꿈으로써 가능하다. 마지막으로, ST-FMR 소자의 $Z_m$은 Vector Network Analyer (VNA) 라는 장비를 이용하여 microwave-network-analysis measurement를 통해 정량적 calibaration이 가능하다. 이에 대해서는 나중에 더 자세히 살펴 볼 예정이다.
대표적으로 두개의 CPW 디자인이 자주 사용되고 있다. Fig. 2(b)에 나와있는 디자인은 asymmetric CPW 이라고 불리며, HM/FM bilayer ST-FMR 측정에서 초기에 사용되던 디자인이다. 하지만, asymmetric CPW는 그 한계가 명확히 존재한다. Fig. 2(d)에 있는 Pt/Py ST-FMR 예제를 고려해보자. 전류에 의해 유도되어 Py 자화에 작용하는 Oersted field ($H_{RF}$)에 더해, 접합 electrode로부터 발생하는 균형이 잡히지 않은 out-of-plane Oersted field가 CPW를 통과하는 $I_{RF}$에 의해 만들어 질 수 있다. 결과적으로 $Hz$ 는 Py 자화에 in-plane torque를 작용함과 동시에, 두 $H_{ext}$방향으로 symmetric voltage signal을 만들어낸다. 결과적으로 스핀류에 의해 생성되는 $\tau_{DL}$이 symmetric signal을 형성하는 과정에서 추가적인 신호가 더해지는 현상이 발생한다. 그러므로, Hz-induced 토크는 Pt의 spin Hall angle의 과대평가, 또는 과소평가를 야기한다. 이 현상은 GSG probe position을 CPW의 중앙에 잘 위치시킴으로써 해결할 수 있다. 이 imbalanced Hz와 이와 관련된 현상을 없애기 위해, symmetric CPW 디자인이 개발 됐으며, 이는 Fig. 4(c)에 나와있다.
4.3. Vector Network Analyzer
앞에서 말했듯이 RF current ($I_\mathrm{rf}$)를 calibration을 하기 위해서 VNA를 이용한 microwave-network analysis measurement를 수행한다. Calibration schematic diagram은 Fig. 3에 나타나 있다. 두개의 똑같은 길이를 가지는 RF cable, 동일한 Bias-Tee, 그리고 두개의 GSG probes를 연결하였고, 이 GSG probe를 standard calibration kit에 through에 연결시켰다. 여기서 우리는 회로의 절반 각각이 동일하게 구성되었으며, power loss나 reflection이 "through"에서 발생하지 않음을 가정한다.
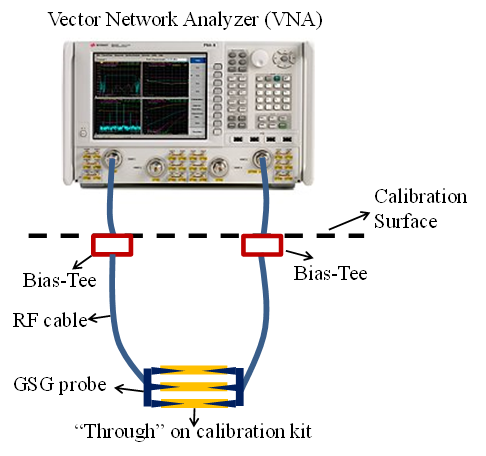
이 셋업을 이용하여, 우리는 trasmission 중에 calibration surface를 measured circuit의 바깥으로 이동시킬 때 Bias-Tee, 1 m의 RF cable, 그리고 GSG probe에서 발생하는 power loss와 reflection을 확인하였다. 예를 들어 ST-FMR 측정중에 cable과 connector에서 발생한 power loss의 총합이 전체 input power의 75%라 하면, 인가한 전력의 25% (13 dBm = 19.95 mW)를 적용하여, 서로 다른 Pt (6 nm)/Ni81Fe19 (Py, 2 ‒ 10 nm) 소자들에서 각각 $I_{rf}$를 계산할 수 있다.
From the measured S11 and S21 parameters, the total power loss in the cables and connectors in our ST-FMR measurement was found to be 75% of the total input power. Thus, using 25% of power (13 dBm = 19.95 mW) in the equation , we can calculate the Irf in different Pt (6 nm)/Ni81Fe19 (Py, 2 ‒ 10 nm) devices, respectively.
We then used the following equations [S1] to obtain the spin Hall angle from the symmetric part of ST-FMR signal separately,
We now have techniques to deal with the few which can
arise such as an additional contribution of fieldlike spin orbit torques to the Oersted
field [49] and the effect of DC spin pumping [31] which we will also briefly
touch upon in Section 3.2.1.
From ST-FMR measurements, τDL and τFL can be separately extracted due to the 90° phase difference in the magnetization dynamics (see section 2.3) [33, 40].
Recalling where each part of the angulardependence originated from, the sin 2 part came from the magnetoresistance,so as long as we are detecting a signal from the same magnetoresistance that part of the angular dependence will be the same. The cos part came from the fact we were assuming the torques were arising from spins polarized in ^ Y , but to consider for example a dampinglike torque caused by spins polarized in ^X we only need to consult Table 3.1 to see that this torque will have a sin angular dependence and will appear as a torque in the ^y direction, and hence will appear on the symmetric part of the lineshape (formy oscillations) by Equation 3.18. With this, we know that the dampinglike torque from spins polarized in ^X will appear almost identically to a dampinglike torque from spins polarized in ^ Y except it will follow a sin 2 sin angular dependence. It turns out that each type of torque (dampinglike or fieldlike) arising from spins in any of the cardinal directions uniquely produce an angular dependence on either the symmetric or antisymmetric signal, and hence can be distinguished by this. So, if we are investigating some new material which breaks enough symmetry to possibly allow torques with arbitrary polarization, we can look at the angular dependence of the STFMR lineshape amplitudes to distinguish and measure them! If the only ^ Y fieldlike torque arises from the Oersted field of the spin source layer, then we can also still use this component as a calibration for all of the other torques since as before the same factors of current and magnetoresistance are shared between the various signals. If this is not the case and there could be other sources of ^ Y fieldlike torques, then extra steps will need to be taken to account for that, either using the method developed by Pai et al. [49] or by attempting to measure the RF current and magnetoresistances directly by some method. Despite this, the analysis of the angular dependence of the STFMR resonance amplitudes is still one of the best ways to analyze torques with complicated polarizations.
The SOT efficiency in Pt has been quantified using different measurement methods by different research groups. However, there have been significant disagreements in the reported values of the SOT efficiency in Pt, ranging from ~0.012 to ~0.12 [32, 51, 52]. To figure out the possible reasons for this discrepancy and determine the intrinsic SOT efficiency value in Pt is of great importance. For ST-FMR measurements, the measured SOT efficiency is evaluated from the number of spins that are absorbed by the FM layer. There are three aspects that affect the measured SOT efficiency. The first is the spin diffusion in the Py layer since the spins can transmit into Py with a characteristic length (i.e. spin diffusion length), which is often ignored. Wang et al [32] carried out Py thickness-dependent ST-FMR measurements in Pt (6 nm)/Py (t = 2–10 nm) bilayers. They found that θsh increases when the Py thickness increases as shown in figure 8(a). By taking into account the spin diffusion in the Py layer, the SOT efficiency of Pt is determined to be ~0.068 at room temperature [32]. The second aspect is spin diffusion in the Pt layer. JS and the measured SOT efficiency θsh in a Pt film of thickness d should be reduced from the bulk value (d =

) by

and

, respectively [25, 32, 45, 59]. For example, the spin diffusion length, λS of Pt estimated from a Pt/Py device is ~1.5 nm [32] (figure 8(b)). The third aspect is the interface transparency as spin currents transmit through the Pt and Py interface, which is discussed in section 5.4.
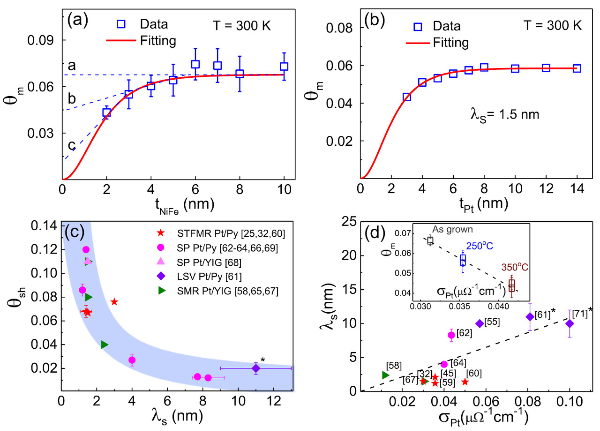
Figure 8(c) shows a summary of SOT efficiencies as a function of λS in Pt measured using different techniques from various research groups [25, 32, 58, 60–69]. A clear correlation between the SOT efficiency θsh and λS is found, which is approximately an inverse relationship with θshλS ~ 0.13 nm (denoted by the blue thick line) [32]. The λS in a material is generally proportional to its electrical conductivity (

) [32, 70]. Figure 8(d) shows an approximately linear relationship between the reported λS and

in Pt films [32, 45, 55, 58–62, 64, 67, 71]. Therefore, the SOT efficiency is inversely related to the conductivity of the HMs. All of the data in figures 8(c) and (d) were measured at room temperature, except for those from [61, 71] which were measured at 10 K, as denoted by small black stars. In addition, it was found that the θsh in Pt remains almost constant as the temperature decreases from 300 K to 13 K [32, 55].
From FMR, we can know
- Magnetic anisotropy
- Magnetic damping
- Spin-wave resonance
From FMR resonance curve, we can know
- Position of the resonance field $H_\mathrm{R}$
- Resonance width $dH$
- The resonance intensity $I$
- # of resonance
Resonance frequency depends on
- The external magnetic field
- The magnetocrystalline anisotropy field
- Shape anisotropy field
- Surface anisotropy
- Defects and stress anisotropy
- Magnetostatic field
Linewidth can be broaden by
- Eddy current
- Joule heating
- Inhomogeniety
- Conductivity
- Porosity
- Strong anisotropic materials
- The presence of ions with strong spin-orbit coupling
Phase Shift Effects
Pt layer 속 $I_\mathrm{RF}$에서 발생하는 Oersted field와 spin Hall torque는 서로가 같은 위상을 가지고 있는데, 이는 이 두개의 field가 같은 RF 전류에 근원을 두기 때문이다. 더욱이, any dephasing between the RF current from the source and within the sample itself is not important. 게다가, Furthermore, we performed the ST-FMR measurement in
the device made from Cu/Py (Ni81Fe19 Permalloy) bilayers, in which Cu is known to have
a very small SHE. As shown in the Fig. 4.11 (measured at 9 GHz) there is a negligibly
small symmetric component (red line) in the ST-FMR signal from Cu/Py, 반면에 Pt/Py로 만들어진 비슷한 소자에서 발생한 ST-FMR 신호는 큰 symmetric signal을 보였다.
(Note that the anti-symmetric components are shown in green). Thus, these control
experiments demonstrate that the phase shift is negligible in our experiments.
The effect of spin pumping
스핀펌핑의 개념은 다음과 같다.
"일관되게 자화 운동을 하고 있는 자성체에 금속을 붙였을때, 스핀류가 자서에로부터 금속으로 주입되는 현상"
즉, 자화의 운동으로부터 전도전자의 스핀류를 만들어내는 것이다.
스핀펌핑은 STT와 온사가르 상반관계에 있는 현상으로서, 만약 자화의 운동이 스핀파에 의한 경우, 스핀파 스핀류가 전도전자 스핀류로 변환된다는 것을 의미할 수도 있다.
이 외에도 자성체 내부의 자구벽 등 완만한 자기구조가 운동하고 있는 경우에도, 자화 다이나믹스가 공간 변화하고 있으므로, 전도전자 스핀류가 발생한다.
불균일한 자화 운동을 하고 있는 자성체 내부에서 스핀류가 발생한다는 사실이 밝혀졌고, 이것을 양자역학의 게이지장을 이용해서 이론적으로 기술하면, 패러데이 전자유도의 스핀버젼이 된다는 사실을 밝혔다.
스핀펌핑의 양자역학적 의미는 아래와 같다.
"시변 자화를 가지고 있는 자성체의 계면에서, 시간 흐름에 따른 반사파의 전자 위상의 변화로 인해 fluctuation이 만들어진다"
이 위상의 시간변화에 스핀 의존성이 있다는 것이 스핀류를 만들어내는 조건이 된다.
앞에서 설명한 것과 같이 전자 위상의 시간변화에 의해 흐름이 발생하는 것을 양자펌핑이라 부르며, 따라서 스핀펌핑은 실온에서 관측 가능한 희귀한 양자펌핑 현상에 해당한다.
스핀펌핑은 다양한 계에서 관측된다. 전형적인 예로, 강자성체/비자성 금속의 이중접합구조에서 막과 평행한 방향으로 자기장을 가한 계가 있다고 하자.
이 경우, 자기장하에서 자성체의 자화는 세차운동을 한다.
이 세차운동의 주기와 같은 주기로 교류자기장을 외부에서 가해 자화를 움직이면, 세차운동의 진폭은 공명이 일어나 매우 커지게 된다(ferromagnetic resonance).
이렇게 아주 큰 세차우동을 하고있는 강자성체에 금속을 붙이게 되면, 자성체에서 금속으로 스핀펌핑에 의해 스핀류가 주입된다. 이렇게 주입된 스핀류는 다음 장에 기술할 역스핀홀 효과에 의해 전압으로 변환하는 것으로 측정이 가능하다.
ST-FMR과 spin pumping 측정에서 발견되는 SOT efficiency는 비슷한 값을 가지며, 이는 다시 말해 이 두 현상이 Onsagar reciprocity를 가짐을 추측할 수 있다. NM에서 발생하는 스핀정보의 근원은 모두 FMR이 일어나고 있는 FM이라고 볼 수 있다. 즉, FM의 입장에선 energy loss로 볼 수 있고, 이는 다시 말해 FMR linewidth broadening을 야기할 수 있다. (Precession (of the spins) is transferred via spin-orbit coupling to the lattice which absorbs thus dampening the resonance.)
Pt layer에서 발생하는 spin pumping에 의한 DC voltage는 symmetrical line shape를 가지며 다음의 방정식으로 표현할 수 있다.

이때 $j_\mathrm{SP}$는 FM에서부터 Pt 층으로 주입되는 spin pumping current이며, effective spin mixing conductance $G_\mathrm{eff}$와 maximum precessing angle에 비례하는 값을 가진다. 식은 아래와 같다. 여기서 f는 RF source의 frequency이다.

또한, 소자의 총 저항은 아래와 같다.
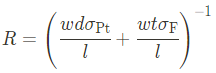
위의 3개의 방정식을 이용하면, $V_\mathrm{SP}$는 아래와 같이 쓸 수 있다.

$\phi$는 실험 결과로서 다음의 공식을 이용하여 구할 수 있다.
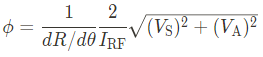
For the experimental results for Pt/Py devices, IRF is calculated to be 6.33 mA for Pt/Py, based on the resistance of the devices (226 for Pt/Py device) and the RF power (14 dbm). is estimated to be 17.6 / rad for Pt/Py, from the AMR (anisotropic magnetoresistance) of Py (0.025), measured on 500 Å thick films. Based on our experimentally determined values of the SHA of Pt (0.19) and the spin diffusion length (1.4 nm), we estimate values of and corresponding to the results in Fig. 4.7, as summarized in the following table. Based on these estimates, we find that the anti-symmetric is much smaller than the symmetric component of the ST-FMR signal (<1% for Pt/Py). Hence, we believe that the spin pumping plays a minor role in our experiments.
ST-FMR 측정에서, spin pumping은 위의 방법이 아닌 inverse SHE에 의해 발생할 수도 있다.
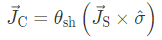
그러므로, 추가적인 DC voltage ($V_\mathrm{SP}$)이 발생할 수도 있으며, 이로 인해 ST-FMR 신호가 오염될 수 이다. Spin pumping contribution은 Pt/Py bilayer의 경우 아래의 방정식을 이용해 구할 수 있었다.

여기서 $\theta_c$(maximum precession cone angle in the device plane)는 아래와 같다.

일반적으로 spin pumping signal $V_\mathrm{SP}$는 ST-FMR의 symmetric component $V_\mathrm{S}$에 비해 한 오더 정도 적은 것으로 알려져 있으며, 또한 $V_\mathrm{SP}$는 소자의 저항에 비례한다. ST-FMR 소자의 저항이 대부분 작기 때문에(~50$\Omega$ for impedance matching), ST-FMR 측정 에서의 spin pumping contribution은 ST-FMR 신호보다 상당히 작은 편이다.
이에 더해, Pt/Py bilayer에서의 SOT efficiency는 Vs/Va ratio와 Vs-only methods 따로따로 정량화 됐으며, 두 방법에서의 SOT efficiency는 크게 차이나지 않는 것으로 나타났다. 이는 다시 말해 Pt/Py 계면에서 발생하는 $\tau_\mathrm{FL}$이 무시할 수준 이라는 점을 암시한다. 하지만, $\tau_\mathrm{FL}$이 Pt/FM 계면에서 Rashba effect로 인해 발생할 수 있다는 논문도 존재한다. 이는 다시 말해 interface의 특징(예를 들어 계면의 quality, 계면의 oxidation 정도)또한 SOT efficiency에 영향을 끼칠 수 있음을 보여준다.다른 한편으로 SOC가 매우 작은 Cu나 다른 insertion layer가 HM과 FM 사이에 삽입되어 interface SOT effect를 제거하거나 줄여줄 수도 있을 것이다.
4.3. Modulation of damping (MOD)
In the context of conventional STT, researchers found that the STT and magnetization fluctuation can be modulated in the vertical MTJs by applying a DC current bias [41, 42]. Recently, this method was used for HM/FM bilayer systems in the spin Hall scheme and the magnetization dynamics can be tuned by applying a DC current in the HM layer [43]. Based on the STT theory [41], the effective magnetic damping (α) and thus the FMR linewidth (ΔH) will increase or decrease depending on the relative direction of spin polarizations with respect to the magnetization direction. The relationship can be written as [25]

, where (ΔH)0 is the linewidth at zero DC bias, (ΔH)sh is the modulated linewidth due to the DC bias induced spin currents, and f is the RF current frequency. Therefore, one can perform the ST-FMR measurements to extract the ΔH as a function of JC as shown in figure 5. By linearly fitting the data, the SOT efficiency is obtained accordingly.
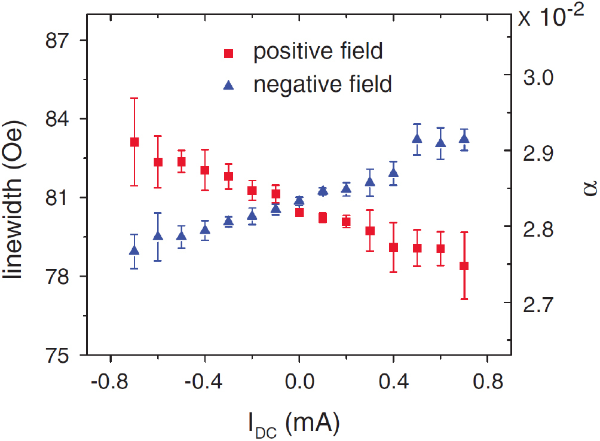
The modulation of damping (MOD) method is an extension of the ST-FMR measurements. So far, it has commonly been used to evaluate SOT efficiency in Pt and Ta [25, 44–47], which usually have considerably large ST-FMR signals. Recently, it has been utilized for the SOT efficiency evaluation of metallic antiferromagnetic materials [48]. However, for material systems possessing smaller ST-FMR signals, it is challenging to employ the MOD method to evaluate the SOT efficiency [40].
Table 1 summarizes the advantages and disadvantages of the above three SOT efficiency evaluation methods. The method of VS/VA is simple; however, it only works for material systems where the VA only attributes to HRF (i.e. there is no significant field-like torque). Therefore, this method has limitations for the SOT efficiency evaluation. However, the method of VS-only can successfully address this issue and can be used as a more general method for extracting the SOT efficiency in a wide range of materials.
Measurement utilizing a vector network analyzer(VNA) instrumentation
- Introduction
시료를 CPW(아래 설명 참고)위에 놓고 자기장을 가하면서 실험을 한다. 이때 자기장의 방향은 x방향이고, microwave의 진행방향과 평행하다. Microwave로 해서 발생하는 섭동 자기은 y방향으로 생성되고, 이 자기장에 의해 시료의 자화방향이 요동하게 된다. 이때 반사되거나 투과되는 microwave를 다시 VNA에서 측정하게 되면, 시료에 의해서 흡수/반사된 microwave를 얻을 수 있고, 이는 자화 감수율의 허수부가 된다. 이와 같이 자기장을 변화시켜가면서 측정한 자화 감수율의 전형적인 예가 그림 6.9에 나타나있다.
- Pros
- 넓은 영역의 주파수를 스캔하면서 실험이 가능하기 때문에 외부 자기장의 변화에 따른 자화 감수율을 독립적으로 측정할 수 있다. 더 많은 측정값을 얻을 수 있어 좀 더 신뢰성 있는 분석이 가능
- CPW(Coplanar waveguide)를 사용하여 microwave를 좁은 영역에 집중시키는 것이 가능하기 때문에 microsize로 패턴된 시료에 대해서도 측정이 가능하다.
- ST-FMR 실험도 가능하다.
- This technique also allos for operation over awide frequency band.
- One varies the microwave freqeuncy at a fixed static field, extracts the FMR absorption profile from standard S parameter measurements, and obtains a FWHM freqeuncy swept linewidth from the response.
- The microwave drive in VNA-FMR is provided by a coplnar waveguide(CPW) excitation.
- The signal analysis is done with a standard vector entwork analyzer.
Frequency swept linewidth from VNA-FMR measurements.
realatively broad bands
- Extracts the FMR absorption profile fro standard S parameter meausrements, and obtains a FWHM frequency swept linewidth 델타f_VNA from the response.
- Conversion of the basic S parameters
- obtain into FMR absoprtion curves and extracted linewidths
'Other Topics' 카테고리의 다른 글
Harmonic Hall Technique (0) | 2023.06.01 |
---|---|
Order parameter dynamics (0) | 2023.05.10 |
Wavepacket (파속) in Quantum Mechanics (0) | 2023.03.13 |
Eigenstates의 확률 진폭의 분포를 나타내는 두가지 방법 (1) | 2023.03.13 |
(작성중) 밸리트로닉스 (Valleytronics) (1) | 2023.03.07 |
댓글